All published articles of this journal are available on ScienceDirect.
Biodecolorization and Biodegradation of Dyes: A Review
Abstract
Dyes are one of the most widely used chemical substances in day-to-day life, including in different industries. Dye manufacturers, as well as users, are experiencing great difficulty in complying with stringent regulations on wastewater containing dyes and derivatives. Most of the industries still use age-old technology and machinery and thus find it difficult to cope with the change in the scenario of current stringent environmental regulations on disposable limits, which are improvised by pollution control boards. The inherent difficulties because of technical inadequacies during dyeing result in a large amount of dyestuff getting directly lost to the industrial effluents. Synthetic dyes are quite stable recalcitrant compounds. Henceforth, the release of dyes poses an ecotoxic hazard and potential danger of bioaccumulation, eventually affecting flora and fauna. Huge quantities of water consumption generate large volumes of highly contaminated effluents. Conventional treatment processes have limitations in the color removal from wastewater. Although physico-chemical techniques are practiced, it still suffers from the ‘economy-to-scale of application’ paradigm and generation of polluting and toxic byproducts, posing disposal problems. In contrast, biological processes involving microbes, plants, or their products (such as enzymes) are touted as alternate cost-effective methods for decolorization and degradation of such synthetic dyes, albeit with limited full-scale successful applications. Biodegradation of such xenobiotics has been the topic of research for over two decades, with limited success because of the production of toxic secondary metabolites and byproducts. This review paper is an effort towards discussing the importance of biodecolorization and biodegradation of dyes, with emphasis on some recent updates such as immobilization techniques and in-silico modelling methods and future possibilities.
1. INTRODUCTION
1.1. Dyes
Dyes are natural or synthetic colored compounds that impart permanent color to fabric, leather, wood, etc. The structural complexity of dyes makes them withstand the harsh conditions and stay ‘bonded’ with the materials they are imparted on. The dyeing history of mankind goes as back as 15000-9000 BC on the walls of Altamira caves in Spain. Inorganic pigments like soot, hematite, ochre, and manganese oxide were used in those drawings. Tyrian purple dye was derived from a marine mollusk found in Lebanon, and its presence, when traced, went back to 1400 BC in the Late Bronze Age [1]. Indigo was another dye used in ancient Roman days. Earlier, this blue-colored pigment was fermentative extracts from Indigofera tinctoria (also known as Anila tinctoria var. normalis Kuntze and Indigo feraanil var. orthocarpa DC). In the thirteenth and fourteenth centuries, natural Indigo was a rare commodity and used to be imported from India. Woad, i.e., Isatis tinctoria (also known as Isatis indigotica Fortune and Isatis japonica Miq), was another source of Indigo, where the Indigofera sp. could not flourish, but the amount of dye that could be extracted from this plant was far less than that could be obtained from the Indigofera plant [1].
Based on their structure, dyes are classified into 14 categories: acid dyes, direct dyes, azo dyes, disperse dyes, sulfur dyes, fiber reactive dyes, basic dyes, oxidation dyes, mordant dyes, developed dyes, vat dyes, pigments, fluorescence or optical brighteners and solvent dyes [2]. Every dye molecule consists of three parts: 1) Chromophores, which are functional groups of dyes, 2) auxochromes, which impart the color, and 3) matrix; the rest of the atoms of the dye molecule comprises this part.
1.1.1. Acid dyes
This is the largest class of dyes that is mainly anionic in nature. The adjective, “acid” refers to the pH of the dye rather than the presence of acid groups in their structures. These dyes are mainly used for dyeing wool, silk, polyamide, and modified acryl [3]. Acid dyes bind to the cationic functional group (NH4+) of the fibers. Acid dyes are mostly azoic (red), anthraquinone type (blue), or triarylmethane type (yellow to green) dyes.
1.1.2. Reactive dyes
This is a type of dye that forms a covalent bond with the ‘–OH’, ‘-NH’, or ‘–SH’ groups of cotton, wool, silk, or nylon fabrics. As the chromophore of these dyes “reacts” with the substrate fiber, these dyes are called reactive dyes. As these dyes form a bond with a wager, too, they are more prone to hydrolysis. That is the reason these dyes have a limited degree of utilization as compared to other types of dyestuff [4]. Despite adding high quantities of fixation agents, almost 40-50% of the reactive dyes do not react with the fiber and remain hydrolyzed in the water phase, leading to colored water effluent. Most of the dyes used in textile industries are known by their trade name and not the chemical name, which poses a serious problem in finding suitable decolorization and degradation methods [5].
1.1.3. Metal-complex dyes
These dyes are not listed as separate categories in Color Index but are mainly acid or reactive dyes with metal complexes. These are complexes of one metal atom with one or two dye molecules, mostly azoic.
1.1.4. Direct dyes
The dyes have a strong affinity towards cellulose fibers and are bound to the fibers by Van der Waals forces of attraction. This is the second-largest group of dyes and is anionic. They can be applied to cotton, linen, silk, rayon, and wool.
1.1.5. Basic dyes
Basic dyes are cationic in nature and are used to dye synthetic fibers such as polyacryl, silk, and wool.
1.1.6. Mordant dyes
These types of dyes need mordant to make the dye fix on the textile and are acidic. These dyes are also used in histology studies for tissue and cell staining. As these are chromium-containing dyes, the wastewater generated after the mordant dyes’ treatment contains high quantities of chromium.
1.1.7. Disperse dyes
These dyes are mainly azo or anthraquinone type and are sparingly soluble in the water. As their solubility is low in the water, they are treated with fiber at high temperatures or in the presence of chemical softeners.
1.1.8. Pigment dyes
These are crystalline, water-insoluble, and non-ionic dyes. These are cheaper options of dyeing and require dispersing agents to dye.
1.1.9. Vat dye
Vat dyes are also insoluble dyes, which are reduced with the help of sodium dithionite and made soluble in water. Ancient Indigo dye is an example of vat dye having a history of over 5000 years. A vat is originally a vessel in which indigo plants were used to be subjected to reduction by fermentation.
1.1.10. Sulfur dyes
These are complex polymeric aromatic heterocyclic sulfur-containing compounds that are insoluble in water. Like vat dyes, dyeing with these dyes also involves reduction and oxidation reactions.
1.1.11. Solvent dyes
Solvent dyes are dissolved in the substrates like plastic, wax, varnish, ink, fat to impart color to them. Most of the solvent dyes are diazo, triarylmethane, anthraquinone, and phthalocyanine in nature.
1.1.12. Fluorescent brighteners
These are the chemicals that are not original dyes but are used to mask the yellowish tint of natural fibers. These compounds lack intense colors and hence are not considered dyes in common. Chemically, fluorescent brighteners are stilbene, coumarin, or 1,2- ethene derivatives. Some of the fluorescent brighteners are pyrazolines, napthilamides, and aromatic or heterocyclic ring compounds.
1.1.13. Azo dyes
This is the largest group of synthetic dyes that has a wide application range in different industries. Azo dyes that contain one N=N are called monoazo dyes, two N=N are called diazo dyes and polyazo, if three or more azo bonds are present in the dye compound. The azo group is generally linked to benzene or naphthalene rings, and it is also possible to be attached to aromatic, heterocyclic, or aliphatic groups [6].
2. ENVIRONMENTAL AND ECOLOGICAL ASPECTS OF DYE USAGE
A huge quantity of wastewater is formed in the textile industry owing not only to the processes in the textile mills but also because of the chemicals used for the processing effluents. A large amount of dyes is wasted in the effluent due to the incomplete absorption of dyes on the fibers and application type. As dyes are designed to be stable against photolytic and chemical degradation, it poses a problem to treat the extra dyes that are flown in the effluent. The accumulation of dyes in the environment, therefore, has ecotoxic hazards, leading to bioaccumulation of these dyes in the food chain. The presence of dyes and high levels of nitrogen (due to high use of azo dyes) in the textile effluents, therefore, needs to be controlled by developing efficient technologies that would allow the increased dye-fiber binding and lower the dye house losses [7]. Diversity in dyes structures makes it difficult to use any singular treatment system to be used for the removal of such a vast array of dyestuff. Generally, the quantity of dyes in the textile wastewater is not significant as compared to the other chemicals, but their intense color poses an aesthetic problem in the disposal. Understanding the chemical structures and developing a strategy to treat the specific type of effluent by the particular chemical and biological method is the plausible option available to textile industrialists.
Extensive use of dyes in different industries such as textile, paper, food, cosmetics, and pharmaceuticals has always encouraged industries to come up with innovative solutions and new types of dyes that can be applied with fewer supplementary materials, such as salt so that the environmental problems associated with it can be reduced. Various demands in textile industries have led to increased use of dyes that are resistant to breakdown by sunlight, water, soap, bleach, or perspiration [8]. The modern textile industry uses dyes that are photolytically and chemically stable to resist the loss of the color of the fabric due to sunlight, water, soap, bleach, perspiration, etc. Furthermore, the use of anti-microbial agents and color mordents have made the color removal from textile effluents very difficult, as they are not easily biodegradable in aerobic conditions. In the textile industry, water consumption varies greatly between a minimum of 0.6 gal/lb for the woven fabric to a maximum of 111.8 gal/lb for felted fabrics [9]. The amount of water needed for a particular textile plant depends on the type of fabric, the type of machine used, types of dyes, and also the type of chemicals used in dye manufacturing and processing units. The treatment process of wastewater from the textile industry depends upon the type of textile manufactured and the types of dyes used in the dyeing process. Furthermore, the process of textile manufacturing, as well as the types of chemicals used in the particular textile mill, also play an important role in the constitution of the wastewater of the mill [10].
3. CONVENTIONAL PHYSICOCHEMICAL DECOLORIZATION PROCESSES
Traditionally, many physical, chemical, and biological treatments are used with a singular application method (Fig. 1). The approach is adopted, yielding low dye removal, low mineralization, and low biodegradability combined with the high cost and need of post-treatment to meet the discharge standards. The combined approach involves the use of physical, chemical, and biological methods in line, yielding better quality effluent at the discharge point. Activated carbons, use of nanomaterials, use of metal ions and composites, and natural materials catalyzed ozonation can be used in a combined way to treat the textile mill wastewater that further leads to a higher percentage of dye removal, lower cost, higher mineralization and biodegradability, COD, and TOC removal percentage is also higher. This approach achieves the complete treatment of textile mill wastewater with a reusability aspect and a higher degree of regeneration [11].
Advanced oxidation processes and biological processes are gaining importance, as these processes lead to the complete mineralization of the pollutants [12]. Advanced oxidation processes involve the use of highly reactive hydroxyl radical formation. Such strong oxidants oxidize the chemical compounds more efficiently than the conventional agents. UV radiation, ozonization, H2O2 treatments, Fenton oxidation process, electrochemical oxidation, photocatalysis, TiO2 photolysis, radiolysis, and wet oxidation processes are to list a few [13]. A combination of these oxidation methods (such as electro-coagulation, electro-oxidation, sono-oxidation, and photo-oxidation) have also been tried and are gaining popularity in the textile wastewater discussion [14]. Other popular methods commonly used are flocculation, coagulation, membrane filtration [15, 16], adsorption [17, 18], and advanced oxidation processes [19-22]. Membrane filtration techniques involve the disadvantage of the generation of chemical sludge that contains insoluble dye particles and starch, besides the regular maintenance of the membranes and initial cost of investment [17]. Combination of UV light and H2O2 is also used as the dye decolorization method, in which a high concentration of hydroxyl radicals produced is responsible for the dye degradation. This method does not further add up to the sludge formation and reduces the foul odor of the effluent. In this method, UV light activates the decomposition of the hydrogen peroxide for the generation of free radicals, which further leads to the chemical oxidation of the dyes and organic molecules, resulting in the formation of end products like CO2 and H2O [23, 24]. Thus, the generation of free radicals is the basic step in this process, which can be achieved by the combination of ozone with hydrogen peroxide in the presence of energy-dissipating components like UV, sunlight, or ultrasound [25]. Such hybrid techniques are comparatively expensive but yield results in less time as compared to other methods when applied individually [26].
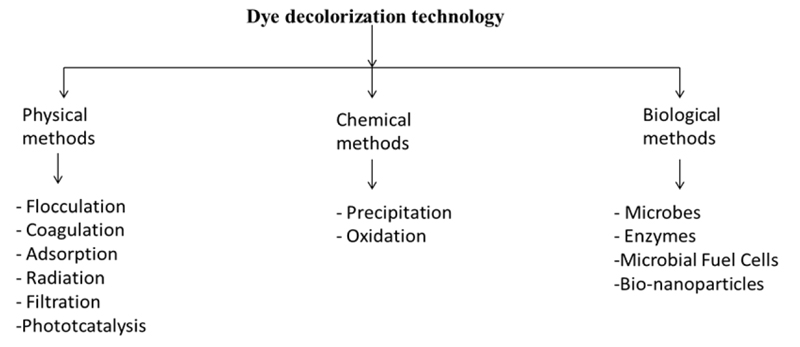
4. BIOLOGICAL METHODS FOR DYE DECOLORIZATION AND DEGRADATION
Aerobic/anaerobic methods involve the use of microbes for the degradation of organic dyes. Microbes or separated enzymes can perform decolorization of dyes [27-39]. Microbial fuel cells are devices that convert chemical energy to electrical energy with the help of microorganisms [40-43]. Specific bacteria having the ability to extract electrons are attached to the anode and carry out the oxidation of the organic matter, releasing protons and carbon dioxide as the end products in the anode chamber. The reduction of oxygen takes place in the cathode chamber, where the electrons generated in the anode chamber are carried by an electrical circuit. This way, microorganisms achieve oxidation of organic matter (such as dyes) and convert the energy into electricity [44-51]. In the last few years, biologically originated nanoparticles are also being used for the photocatalytic degradation of the dyes [52-61].
4.1. Recent Advancements in Biodecolorization and Biodegradation of Dyes
Dyes are highly persistent in natural environments, as these chemicals were intended to be chemically and photolytically stable [62, 63]. Their release into the environment is potentially hazardous, as it involves ecotoxicity risk and can affect the food chain [64]. Water-soluble dyes like acid, reactive, disperse, and basic dyes have less bioaccumulation [64]. It is reported that ionic dyes have the least bioaccumulation potential (but some acid dyes may bioaccumulate), whereas non-ionic dyes and pigments, however, have a high bioaccumulation potential [65]. Unlike physicochemical methods of dye decolorization, biological methods are relatively economical, more efficient, environment-friendly, and more versatile [66]. Using biological agents such as microorganisms has considerable advantages over the traditional methods. The processes are relatively inexpensive and lower running costs. Moreover, this method also offers complete mineralization of the organic pollutants [67].
Decolorization of dyes by microorganisms may take place either by adsorption of dye molecules on the microbial biomass or degradation of dyes by microbes [68]. Azo dyes degradation is often carried out using consortia of microbial culture. Co-metabolic activities within the microbial community in the consortia help to bring about a higher degree of biodegradation and mineralization. The group of microorganisms in the consortium together is reported to carry out the biodegradation of difficult substrates efficiently as compared to individual pure strains. Such mixed-culture studies would provide a better comparison to real-world scenarios. Although several biodegradation studies reported better biodegradation efficiency, the results are often not easily reproducible, which makes it difficult to understand the mechanism. Henceforth, the majority of studies have been carried out and reported using pure-individual microbes. Using pure cultures ensures the reproducibility of the data, and concrete interpretations can be drawn based on such studies. Azo dyes are decolorized with the initial anaerobic azo bond reduction, which results in colorless compounds. The resultant aromatic amines are then degraded under strict aerobic conditions. Thus, the complete mineralization of the azo dyes involves a combination of anaerobic/aerobic processes. Yet, the selection and isolation of bacteria having both aerobic and anaerobic survival and degradation properties are a difficult task. In the recent past, different enzymes (such as azoreductase, laccases, catalases, oxidases, and peroxidases) are reported for decolorization and degradation of dyes, as mentioned in Table 1. Decolorization of textile dyes using bacterial or fungal cells can be achieved through living as well as dead cell mass [66]. This biosorption may be of interest due to the recovery of intact dyes using suitable solvent systems from these cell masses, but the process itself is difficult for practical use. Also, the sludge disposal problem is one of the major hurdles to the practical application of this method. In the biodegradation process, however, organic dyes are degraded into fragments that might themselves be xenobiotic, which further need to be completely mineralized into CO2 and H2O. The biodegradation process seems more natural and has the potential to eradicate the existence of these organic pollutants.
Enzyme | Producing Species | Dye | Decolorization/Degradation Efficiency (%) |
---|---|---|---|
Microbial origin | |||
Azoreductase | Bacillus pumilus, Lysinibacillus sp. KMK-A, Nostoc lincki, Scenedesmus bijugatus, Chlorella vulgaris, Haematococcus sp. | Remazol blue, Orange M2R, Methyl red, Tartrazine, Direct blue 71, Direct red 23, Direct orange 26, Congo red | 68-100 |
Laccases | Escherichia coli K 12, Pseudomonas extremorientalis BU118, Streptomyces ipomoeae CECT 3341, Bacillus thuringiensis RUN1, Pleurotus sajor-caju, Trametes trogii SYBC-LZ, Paraconiothyrium variabile, Trametes versicolor, Aspergillus sp., Trametes versicolor, Peroneutypa scoparia, Oudemansiella canarii, Phomopsis sp. | Congo red, malachite green, Remazol Brilliant Blue R, Indigo carmine, Malachite green, Reactive black-5, Remazol Brilliant Blue R, Acid red 18, Sulphur blue 15, Reactive black 5, Reactive blue 114, Reactive blue 19, Acid red 97 | 75-98.5 |
Mixture of different enzymes (Laccase, lignin peroxidase, tyrosinase, azoreductase, NADH–DCIP reductase, manganese Peroxidase, Veratryl alcohol oxidase, Tyrosinase) | Alcaligenes faecalis PMS-1, Pantoea ananatis, Bacillus fortis E4 Pb3, Alcaligenes faecalis, Brevibacillus parabrevis strain GRG, Bordetella trematum, Phanerochaete chrysosporium CDBB 686, Climiddleilus scyphoides, Ganoderma rasineceum, Sterigmatomyces halophilus SSA1575 | AB193, AB194, Congo red, Poly R-478, Methyl green, Bromophenyl blue, Bromothymol blue, Remazol brilliant blue R, Reactive Black 5, Reactive orange-13 | 41.84 - 100 |
Peroxidases (Manganese peroxidase, Lignin peroxidase | Irpex lacteus F17, Ganoderma lucidum IBL-05, Curvularia clavata NZ2, Coelastrella sp., Trametes pubescens strain i8 | Malachite green, Sandal-fix black CKF, Congo red, Remazol Brilliant Violet 5R (RBV5R), Direct Red 5B (DR5B), Triphenylmethane dye, Poly R-478, Remazol Brilliant Blue Reactif | 80 - 96 |
Catalase | Dermatocarpon vellereceum | Navy blue HE22 | 98 |
Plant origin | |||
Peroxidases/Lignin peroxidase | Saccharum spontaneum, Ipomea palmate, Momordica charantia, Brassica rapa, Glycine max, Luffa acutangula, Ammannia beccifera, Fimbristylis dichotoma, Ziziphus mauritiana, Brassica rapa, Zingiber sp. | Supranol green, Procion green HE-4BD, Supranol green, Disperse red 17, Reactive blue 21, Methyl orange, Direct red 23, Crystal Ponceau 6R, Reactive Blue 4 | 57 - 100 |
Laccase | Rhus vernificera, Blumea Malcolm ii Hook | Reactive red 120, Brilliant Blue R | 90 - 98 |
Polyphenol oxidase | Cydonia oblonga | Telon yellow ARB | 72.68 |
Lignin peroxidase, tyrosinase, DCIP and Azoreductase | Blumea Malcolm ii Hook | Malachite green, red HE4B, Methyl orange, Reactive red 2, Direct red | 76.50 – 96.61 |
Lignin peroxidase, catalase, veratryl alcohol oxidase | Salvinia molesta | Rubine GFL | 97 |
4.2. Factors Affecting Dye Biodegradation
Biological treatment systems are highly variable, and in textile effluents, there are several factors that can affect the biodegradation of the azo dyes. Temperature, pH, aeration, the type of reducing agents used, microbial consortium, etc., are the factors that can affect the biodegradation of dyes. Further, the type of dye, the functional groups of the dye, its concentration, additives such as mordants, and their concentration in the effluent are also important while considering the wastewater treatment strategy. Azo dyes are not readily degraded in aerobic conditions, although the specialized enzymatic machinery for aerobic degradation of certain azo dyes has been reported [76]. In anaerobic systems, azo dyes are degraded using non-specific azoreductase enzymes and are converted into aromatic amines that may or may not be degraded in anaerobic systems. Subsequent aerobic biodegradation of the aromatic amines is necessary, as the accumulation of these amines can prove lethal to the biological system of the treatment plant. Studies show that several bacteria are capable of dye decolorization. The enzymes involved are mostly azo and nitro reductases that depend on one or the other co-factors, such as NAD or FAD, for their catalytic activity [77]. Other classes of constitutive enzymes, such as catalases and peroxidases, which are present in bacteria, also can degrade azo dyes [78]. Several bacterial systems have been observed to degrade dyes and take part in the recalcitrant decolorization process. Various environmental factors, such as pH, temperature, salinity, cations, anions, BOD, COD, and oxygen, affect the biodegradation of pollutants in natural ecosystems. Hence, optimization of physical and environmental parameters is important to increase the dye decolorization efficiency of the organism.
4.3. Immobilization of Microbial Cells/Enzymes for Improved Decolorization Efficiency
Immobilization of cells has been defined as imprisonment of microbial cells or their enzymes in the distinct phase that allows exchange with but is separated from the bulk phase, in which the substrate, effector, or inhibitor molecules are dispersed and monitored. Entrapment of cells within the biopolymer matrix is easy to perform. The cell suspension is mixed with the solution of polymer precursor, and polymerization is initiated. Generally, two types of polymers are used: polyacrylamide-type gels and naturally derived gel material like gelatin, cellulose triacetate, agar, alginate, etc.
Several bacterial strains degrade and detoxify a variety of dyes, where whole-cell immobilization is one of the promising methods to increase the efficiency and stability of such cells. The immobilization process supports a high cell density and applications in semi-continuous or continuous mode in reactors. The catalytic ability is often seen to be improved upon immobilization. Immobilization is reported to enhance tolerance to toxic substrates and helps degrade higher concentrations of toxic compounds as compared to non-mobilized microbial cells [79]. Immobilization also permits reuse of the same culture for decolorization of several batches of the effluent and generating comparatively less amount of sludge. Hence, it is economically more sound than the use of free bacterial cells. Immobilization has many advantages over traditional free cell systems, like continuous utilization of the substrate, reduction of interfacial interaction, stimulation of production and excretion of secondary metabolites, protection from the high shear environment, and high decolorization speed. In addition, cell immobilization helps in maintaining high biomass concentration. Cells are protected from the toxic effects of dye and its by products. Moreover, the method is cost-effective, and the same cell culture can be reused multiple times [80]. Enzyme immobilization enhances the efficiency of the catalytic activity and storage and operational constraints are also improved; also, enzyme recovery and reusability are the added advantages. Even though several reports highlight enzyme immobilization studies (Table 2), their applications in the industries are still limited because of the restriction of mass transfer, scaling up and continuous operations difficulties, and difficulty in separation of immobilized enzymes from reaction mixtures [81].
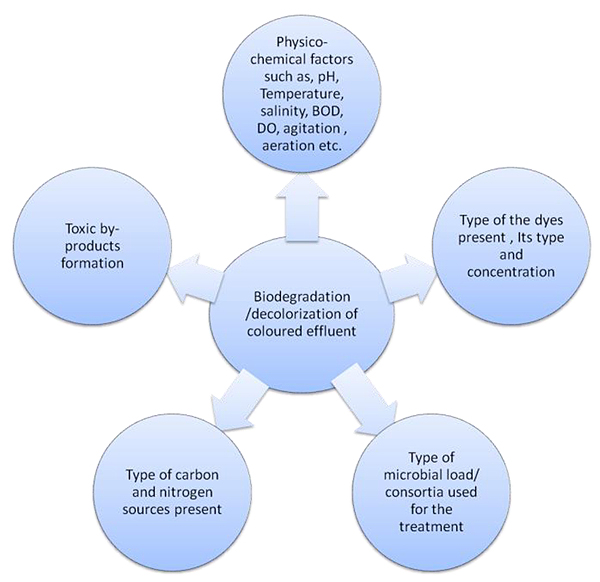
Dyes | Enzyme/Microorganism | Carrier/Matrix/Conjugate | Source of Waste/Experimental Conditions | Decolorization/Degradation Efficiency % | Reference |
---|---|---|---|---|---|
Disperse, direct and reactive dyes | Manganese peroxidase | Ca-alginate beads | Sitara Textile industry effluent, Faisalabad, Pakistan; in the presence of H2O2, 1-hydroxybenzotriazole, pH (5.0), 40 °C, 5 h incubation | 87.4 | [82] |
Reactive Blue 19 | Coriolus versicolor laccase | Bacterial nanocellulose | Horizontal rotating reactor and a traditional vertical mixing reactor; 55°C; pH 4.0; agitation speed 20 rpm | 45.7 – 88.2 | [83] |
- | Phanerochaete chrysosporium Manganese peroxidase | Glutaraldehyde activated chitosan beads | Crescent Textile industry effluent, Faisalabad, Pakistan | 97.31 | [84] |
Remazol Brilliant Blue R, Reactive Black 5, Congo Red, and Crystal Violet |
Horseradish peroxidase | Chitosan beads | Packed bed column bioreactor/Effective up to six decolorization cycles | 82.17 – 97.82 | [85] |
Direct Red 23, and Acid Blue 92 | Laccase | Graphene oxide nanosheets | A 250 mL total volume, 150 mL working volume; 45 °C; Effective up to six decolorization cycles | 75 | [86] |
Congo red | Laccase | Chitosan nanoparticles on the surface of activated glass beads | 15 min incubation; 40 °C; nanoparticulated immobilized enzyme was retained even after 25 successive cycles | 98 | [87] |
Sandal reactive dyes | Lignin peroxidase from Ganoderma lucidum IBL-05 | Ca-alginate beads | Orbital shaker (120 rpm); 3 days; 30°C | 80 - 93 | [88] |
Methyl orange | Horseradish peroxidase | Polyvinyl alcohol-alginate beads | At 30 °C; 150 rpm; Immobilized-HRP retained 64.14% of its initial activity even after 10 consecutive cycles | 100 | [89] |
Five different textile reactive dyes | Trametes versicolor IBL-04 laccase | Chitosan microspheres | Incubation at 25 °C; 4 h; rotary shaker (120 rpm); Retained 80.19% of its activity after ten continuous decolorization cycles for Sandal-fix Red C4BLN | 100 | [90] |
Azo dye acid orange 7 | Coriolus versicolour (NBRC 9791) | Spongy pellets and attached biofilm | Membrane bioreactor | 95 - 99 | [91] |
Congo red | Bacillus sp. MH587030.1 | Polyurethane foam-polypropylene | Moving bed biofilm reactor | 95.7 | [92] |
Reactive Brilliant Blue X-BR, Remazol Brilliant Blue R, Congo Red, Acid Black 172, Methylene Blue, Neutral Red, Indigo Blue, Direct Fast Blue FBL, | Trametes pubescens laccase | Chitosan beads | Retained 60% of its activity after ten continuous decolorization cycles | 20.81 - 68.84 | [93] |
Commercial aromatic dyes | Laccase from Ganoderma sp. KU-Alk4 | Copper alginate | Five liter capacity airlift bioreactor | 100 | [94] |
Alizarin red S | Laccase | Transition metal oxides nanomaterials | Photodegradation at 31.1 °C, and light intensity 452 ± 183 W/m2 | 85 - 95 | [95] |
Reactive yellow dye (RY15) | Mixed microbial consortia isolated from activated sludge | Sodium alginate, starch, and Gelatin, by the cross-linking with polyvinyl alcohol | Sequential anaerobic–aerobic process in bench-scale bioreactors and lab-scale bioreactors | 100 | [96] |
Reactive Blue 4 | Ginger peroxidase | Polypyrrole-cellulose-graphene oxide nanocomposite | It retained approximately 72%of its initial activity even after 10 sequential cycles in batch process | 99 | [97] |
Malachite green, brilliant green, crystal violet, azophloxine, Procion red MX-5B, and reactive blue 19 | Laccase | Fe3O4@C-Cu2+ nanoparticles | It retained >61% of its original activity even after 10 consecutive reuses | 75 – 99 | [98] |
Direct blue 15, Evans blue, Reactive black 5 and Acid red 37 | Laccase from Weissella viridescens LB37 | Magnetic chitosan nanoparticles | It retained ~47% relative activity even after 10 cycles | 93 - 97 | [99] |
Microorganism/Enzyme | Dye | Docking software/details | Decolorization efficiency | Reference |
---|---|---|---|---|
Tropical mangrove actinomycete, Streptomyces coelicolor strain SPR7 | Methylene Blue | Autodock software to discern docking score, binding pocket residues and interaction of azoreductase, DyP-type peroxidase and laccase with dye | 97.5% within 72h | [103] |
Aeromonas hydrophila SK16 and Lysinibacillus sphaericus SK13 (laccase and azoreductase enzymes) | Reactive Yellow F3R, Joyfix Yellow 53R, Remazol Red RR, Drimaren Black CL-S and Disperse Red F3BS |
Homology models using Accelrys Discovery Studio 3.5; Docking using LeadIT software for binding energy calculation, and active site residues identification | 90.4% | [101] |
Aeromonas hydrophila MTCC 1739 and Lysinibacillus sphaericus MTCC 9523 | Textile azo dye Drimaren Red CL-5B | Biosolve IT software to calculate docking score, identify active site residues and molecular interactions between laccase and azoreductase with dye |
88.35 – 91.96% | [102] |
Purified recombinant bacterial laccases | Rose bengal, malachite green, and congo red |
Molecular dynamics (MD) simulations using Gromacs version 4.5.5 with the gromos96 43a force field |
74.5 – 90.4% | [104] |
Laccase and azoreductase of Aeromonas hydrophila and Lysinibacillus sphaericus | Reactive Red F3B, Remazol Red RGB, Joyfix Red RB, Joyfix Yellow MR, Remazol Blue RGB and Turquoise CL-5B |
In-silico docking tool BioSolveIT-FlexX | 9 – 96.07% | [105] |
Klebsiella sp. KOD36 | Azo dyes | Simultaneous aerobic biodegradation of azo dyes and hexavalent chromium was modeled using machine learning programming (using gene expression programming, random forest, support vector regression, and support vector regression-fruit fly optimization algorithm) |
94 – 97% | [106] |
4.4. Systems Biology, in-silico Molecular Modeling, and Docking studies for Dye Decolorization
Recent systems biology approaches integrate various molecular (homology) modeling, molecular docking, metabolomics pathway analysis, and structure-based inhibitor design and cellular behavior [100, 101]. The selection of the method and technique depends on the type and amount of available data. For example, molecular docking method could aid in predicting the interaction between enzymes (amino acids) and dyes for better-efficient dye decolorization strategies by predicting different ‘protein-ligand-inhibitor’ parameters to efficiently transfer the technology for scaled-up applications [101]. It could help to predict and screen different polluting dyes and the possibility of being remediated by enzymes. Philem and Adhikari [100] identified novel target genes that could increase azoreductase activity to accelerate azo dye degradation by a Cyanobacterium nostoc sp. PCC7120, using bioinformatics tools. Such in-silico approaches could help to predict the nature of the contaminant and biodegradation pathways by microorganisms (or enzymes) capable of biotransformation (and possibly biodecolorization and biodegradation). Even though it is somewhat difficult to screen all the different experimental approaches for dye decolorization methods, it is strongly recommended to experimentally confirm the efficiency of any possible interactions after such in-silico techniques for better bioremediation [101]. In short, such in-silico molecular modeling and docking studies can assist as a primary screening process (whenever the information is available for such microbes and/or enzymes) to study a potential technique, which could be employed for cost-effective dye decolorization and degradation applications [102]. Some recent studies are highlighted in Table 3.
CONCLUSION AND FUTURE PROSPECTS
Chemical dyes were synthesized to be stable and persistent compounds, making them widely used chemicals and some of the most studied recalcitrant compounds and pollutants. Although they could be treated or degraded by different types of physico-chemical treatment methods, biological methods are more desirable due to several environmental benefits [107]. In general, microbes (bacteria, fungi, and algae) and plants could be used, or their enzymes are widely applicable for dye adsorption, catalysis, decolorization, and degradation applications. Biological catalysts, i.e., enzymes, have been widely studied and reported to be environmentally friendly and economical. Several types of oxidoreductase enzymes (from both microbial and plant origin), such as laccases, azoreductases, oxidase, and peroxidases, showed excellent performance, both at lab-scale studies and real applications in the field. These enzymes are easily available from different sources, quite stable, and effective under a wide range of environmental conditions (temperature, pH, and salinity). Some reports suggested that the mixture of different types of enzymes could provide better decolorization efficiency as compared to individual enzymes. Recent advances in enzyme immobilization using different types of biological agents or nanoparticles showed higher degradation efficiency and better stability and reusability. However, co-generation of toxic intermediates is one concern of enzymatic degradation processes and needs to be studied properly. Several factors should be optimized and analyzed before field-scale applications of such techniques. Our understanding of recent OMICS and systems biology, in-silico molecular modeling, and docking studies have a great potential for dye decolorization applications. Such in-silico studies will benefit in designing tailor-made treatment options in the future for the treatment of such recalcitrant pollutants.
CONSENT FOR PUBLICATION
Not applicable.
FUNDING
None.
CONFLICT OF INTEREST
The authors declare no conflicts of interest, financial or otherwise.
ACKNOWLEDGEMENTS
The authors would like to kindly acknowledge the support provided by S. P. College, Pune, India, and Sultan Qaboos University, Oman.