All published articles of this journal are available on ScienceDirect.
Phytotoxicity of Nanomaterials in Agriculture
Abstract
Science and technology have advanced rapidly in every aspect; thus, nanotechnology is one of the highly promising interdisciplinary approaches which has swiftly emerged in the world. The inherent properties of nanomaterials (NMs) made them widely accepted to use in many fields, including agriculture. Because of this, NMs have attracted novel agrochemical formulations to enhance crop productivity. However, deliberate and accidental release of nanoparticulate based agrochemical formulations and engineered NMs have raised concerns on the possible effects on agricultural crops. Therefore, the interaction of NMs leading to phytotoxicity is the biggest concern that is required to be assessed prior to their applications. Hence, this review discusses whether NMs can be used as a feasible stand-in candidate for agriculture.
1. INTRODUCTION
The development of nanotechnology has touched the quality of life in almost all stakeholders providing several products and benefits to the society, environment and economy [1]. Nanotechnology utilizes particles with at least a single dimension between 1-100 nm for these applications, where it is simply known as nanoparticles (NPs). Therefore, unique and novel properties of NPs enable to use them in various applications in medicine, energy, food industry, environmental remediation, electronics etc [2-7]. Also, agricultural sector possesses a great interest in using nanomaterials (NMs) to overcome the issues causing loss of soil fertility [8]. Therefore, as an interdisciplinary approach, nanotechnology has enabled to expand its applications over a range of fields.
Although the use of nanotechnology has gained a significant interest, the nonregulated use of NPs has caused several problems, challenges and consequences to living organisms and ecosystem balance [6]. The release of NMs derived from natural and anthropogenic sources raised several concerns on their potential toxicity. The NPs are released to the environment through the processes such as photochemical reactions, volcanic eruptions, forest fires, simple erosions as well as from plants and animals [9]. Furthermore, agricultural applications, atmospheric depositions, rain erosion, surface runoffs lead to the entry of NPs into the soil. Reports have shown that weak migration ability of NPs in the soil leads to more accumulation in high concentrations than in water or air. Therefore, soil can be regarded as the major compact where NMs will end up [10, 11]. Hence, the deliberate or accidental release of NMs to the environment has intensified the questions regarding their use and effects on the ecosystem.
Plants as primary producers play a critical role in any community by harnessing solar energy, especially to carbohydrates and providing energy for other trophic levels [11]. Plants were evolved in the presence of natural NPs, but the present use of engineered NMs has enhanced their probability of exposure. Hence, direct and accidental release of NMs from contaminated soils and sediments or atmospheric fallouts results in the accumulation of NMs in plants [12].
The interaction of NMs with plants may show positive as well as negative impacts on plants. The capability of NMs to bioaccumulate, biomagnify and transfer via trophic levels led to make more concerns on their potential toxicity to plants. Because plants are the pioneers of fulfilling the food requirement of organisms either via direct or indirect modes, any harm to this trophic level will adversely affect other trophic groups [11-13]. Therefore, assessing the NMs as potential candidates for agricultural applications is imperative.
2. FACTORS AFFECTING THE TOXICITY OF NMs
The simplest explanation to understand the fate of NMs against biological systems is to study their physicochemical properties and the interactions with the environment [14]. Physicochemical properties include size, shape, composition and surface chemistry of NPs and these properties will determine their effects on living components. Primarily, NPs can be grouped according to their dimensionality, morphology, composition, uniformity and agglomeration properties. The dimensionality of NPs plays a critical role in its function, especially in their potential effects. Based on its dimensionality, NPs can be categorized as one-dimensional (1D), two-dimensional (2D) or three-dimensional (3D). Moreover, morphological characteristics, including flatness, sphericity, and aspect ratio can enhance their properties. Also, chemistry and electromagnetic properties allow the NPs to exist as dispersed aerosols, suspensions/colloids or in agglomerated states [14, 15]. Despite different classifications, NPs are generally classified based on their composition [9, 16, 17] as carbon-based nanomaterials (CNMs), metal based NMs, dendrimers and polymers, bio-inorganic complexes and quantum dots, etc.
Furthermore, interaction with the environment can alter their chemical properties and reactivity. Hence, this can lead to aggregate NMs forming complexes with organic matter and colloids in soil and water thereby, severly influencing bioavailability by abiotic factors [9, 14]. Moreover, same NM has different effects on different model plants. For instance, TiO2 NPs on Spinacia oleraces show a 60% increment in plant fresh and dry weight. In contrast, the same NM at the same concentration tested on Zea mays shows a growth inhibition [18]. Therefore, the model plant selected for a study plays an important role in the phytotoxicity of NPs as well.
3. PLANT UPTAKE OF NPs
The NPs can be dispersed in different environmental matrices, in which their interaction can be extended from root to leaves [14]. Therefore, NPs accumulated in the atmosphere can directly interact with aerial parts of the plants where they can enter into plants through stomatal pathways, through plant stems and through other plant cellular channels [19]. In contrast to accumulation in the atmosphere, NPs can be frequently found in other environmental matrices such as water and soil. Hence, uptake of different NMs into plants occurs through its root systems via selective uptake and NPs even undergo biotransformation within the roots. Eventually, NPs may translocate to different parts of the plant [12, 20, 21].
In comparison to bulk counterparts of NMs, the physicochemical properties of NPs enhance their reactivity to their environs, thus leading to interaction with plant root exudates and peculiar membrane transporter. Studies have exploited three different means of NP penetrations into plant cells based on the size, shape, charge, hydrophobicity, chemical composition and stability as mentioned elsewhere in the text [22]. Direct diffusion of NPs through the phospholipid bilayer is regarded as the first mechanism. Secondly, penetration via endocytosis in which plasma membrane develops deformed inwards around the NPs, where this will invaginate to surrounding NMs followed by internalized vesicles in the cells. The third mechanism involves ion channels and aquaporin. This mechanism is limited by the selectivity and small pore-sizes. Also, the establishment can be induced by the mechanical action of NPs and it is observed in the cell membranes [23].
Once NPs are internalized, they can be transported to different parts of the plant via apoplastic or symplastic pathways, via xylem vessels and crossing the plasmoderms. However, the efficiency of uptake and translocation is species-dependent where it may be based on the physiology of the plant species [14, 24]. In addition, many studies have exploited the characteristics of NPs, such as size, surface charge, mobility and dissolution within the biological system play a critical role in internalization within plants [25]. However, more studies are in need to better understand the behavior of NMs under realistic conditions.
4. PHYTOTOXICITY OF MOST WIDESPREAD NPs
Many reports available on the interactions between plants and NPs include the metallic NPs, CNMs and Silica NPs. Most of the studies have reported on metallic NPs because of their ease of absorption by roots and the ability to provide essential micronutrients in easily accessible form to plants [8, 14]. It is noteworthy to point out that the interactions of NMs with plants may show positive as well as negative impacts depending on the physicochemical properties. Hence, Fig. (1) has summarized the effects and interactions of NMs with plants. Therefore, some of the most utilized NPs as nanofertlizers, and their phytotoxic effects on different plant systems as well as the bioassays to assess the phytotoxic effects are reported here.
4.1. Plant Bioassays
Phytotoxicity studies are usually performed using well-established plant bioassays, which will allow screening and monitoring the environmental contaminants by analyzing the relation between the dose applied and the extent of induced damage in plants [26]. Ideal plant bioassays include easiness of handling, low cost, high sensitivity, and good correlation with the results of animal assays. Model plants include dicotyledons, such as Vicia faba L. and Allium cepa L. and monocotyledons; Hordeum vulgare L. and Zea mays L. Furthermore, edible plants such as ryegrass, zucchini, lettuce, radish crop plants and wheat have also been used in plant assays, especially on NMs [27]. The tests include a range of endpoints as physiological and morphological parameters and may evaluate the effects on mitotic activity, cell cycle (cytotoxicity), and DNA damage caused by clastogenic and mutagenic activity (genotoxicity) after an exposure period to assess acute or chronic damage [14].
4.2. Effects of Metal and Metal Oxide- Based NMs
A number of studies has been carried out on the phytotoxicity of metallic NPs. Metallic NMs such as Zn, Cu, Fe, Mn, Ag and their oxides, including TiO2, Al2O3, CuO, Fe2O3 is widely exploited for their phytotoxicity in different model plants [8, 28-35].
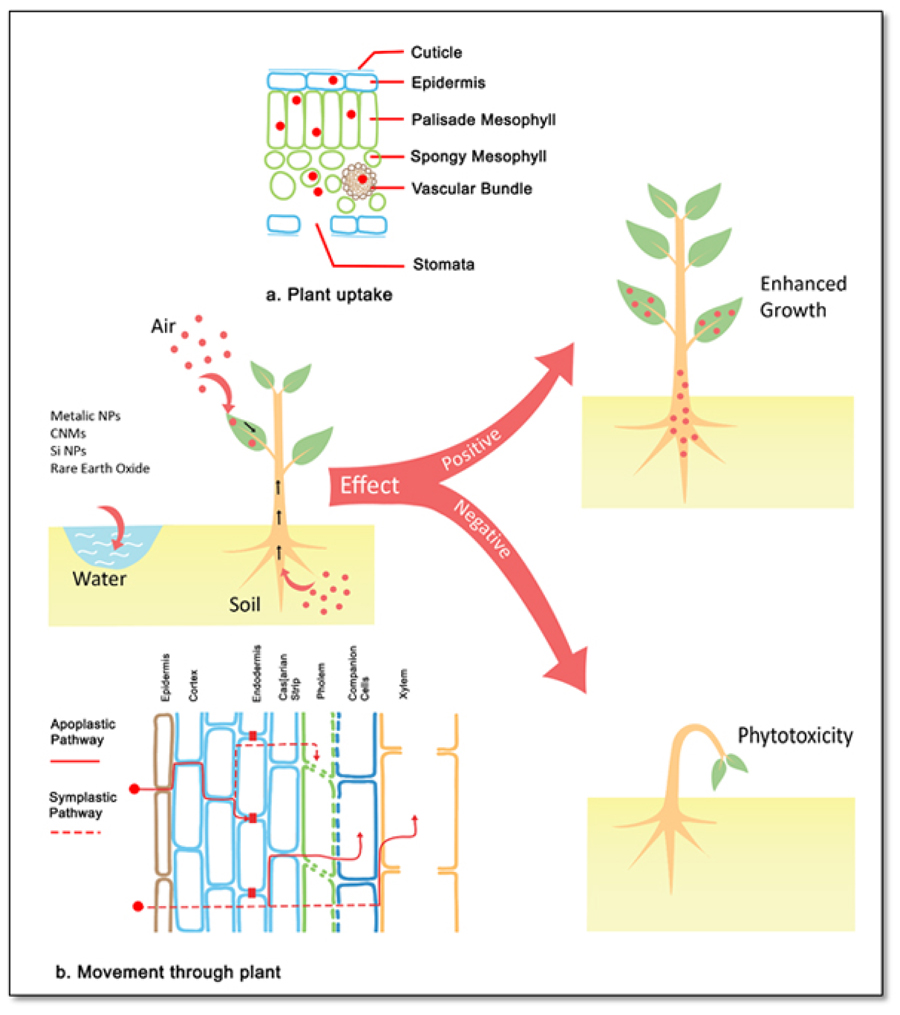
4.2.1. Effects of Copper Oxide NPs
Rajput et al. [36] observed that CuO NPs inhibit the growth by affecting the shoot and root elongations, maximal quantum yield of photosystem II, and transpiration rate of Hordeum sativum. It was speculated that the suppression of photosynthesis might be due to decreased electron transport, the number of thylakoids per granum, photosynthetic rate, transpiration rate and stomatal conductance. Phytotoxicity of CuO NPs of size 43 nm in the presence of humic acid was reported by Peng et al. [37]. The authors reported that CuO NPs had induced the inhibition of root elongation, abnormality in root morphology, and its ultrastructure. Further, cell viability and membrane integrity were also reported. These adverse effects had resulted from the generation of reactive oxygen species caused by CuO NPs, which led to mitochondrial dysfunction, lipid peroxidation, and programmed cell death in rice seedlings.
4.2.2. Effects of Iron and Zinc Oxide NPs
Treatment of Fe2O3 NPs at 50 mg/L concentration has decreased root elongation of Lactuca sativa by 20%, compared to control plants [38]. More recently, Sun et al. [39] reported that Allium cepa plants treated with 5 and 50 μg/mL ZnO NPs showed cytotoxic and genotoxic effects in the root meristems by affecting the cell membrane integrity, metabolic activity, reactive oxygen species accumulation, DNA damage, chromosome aberrations and cell cycle progression. It was found that ZnO NPs treatments accounted for 24.2 or 36.1% root inhibitions when the plants were exposed to 36 h period.
4.2.3. Effects of Titanium dioxide NPs
Hu et al. [40] depicted that TiO2 NPs with 400 mg/L concentration show a negative impact on the nutritional quality of Lactuca sativa. Exposure of Oryza sativa to higher concentrations of TiO2 NPs (1000 ppm) led to accumulating higher amounts of Ti in roots than in shoots. Significant reduction in CO2 fixation, transpiration rate and stomatal conductance was reported on tests. In addition, insignificant effects on photosynthetic pigments, quantum efficiency of PSII (Fv/Fm ratio) and photochemical quenching were also exploited by DaCosta and Sharma [41]. Frazier et al. [42] investigated the effect of TiO2 NPs on tobacco and found that higher concentrations of NPs could significantly inhibit the germination rates, root lengths, and biomasses of tobacco seedlings. Similarly, TiO2 NPs significantly influenced the expression profiles of microRNAs (miRNAs) that have been shown to play an imperative role in plant development as well as plant tolerance to abiotic stresses such as drought, salinity, cold, and heavy metal was also highlighted by authors.
4.2.4. Effects of Silver NPs
The Ag is another group of metallic NPs with wide use in different fields; however, Rui et al. [43] reported that soil amendment of Ag NPs on peanut cultivars (50, 500, and 2000 mg/kg) for 98 days had shown a significant reduction in plant growth and crop yield at the concentrations tested. Furthermore, the fatty acids in the grains of peanuts indicated the presence of Ag NPs could significantly alter the crop quality. In another study, Li et al. [44] point out that the possibility of contaminating fruits, seeds, and other edible parts by translocation processes in plants exposed to AgNPs.
In contrast, the same NMs show promising results in agricultural applications as potential candidates as nanofertilizers. Ghafariyan et al. [24] reported that low concentrations of superparamagnetic Fe-NPs (0-2 mg/L) significantly increased the chlorophyll contents in sub-apical leaves of soybeans under hydroponic conditions, proving that soybean could use this type of Fe-NPs as a source of Fe. The cucumber plants grown in soil treated with ZnO NPs at 400 and 800 mg/kg showed that at 400 mg/kg ZnO NPs had significantly increased the starch content was reported by Zhao et al. [45]. Green synthesized AuNPs using onion extracts on onion seeds were evaluated by Acharya et al. [46]. It was exhibited that applying AuNPs as priming agent at low concentrations (5.4 ppm) resulted in enhancement of germination, plant height, leaf length, leaf diameter, neck diameter, and leaf surface area. Moreover, Sathiyabama and Manikandan [47], speculated, both foliar spray or as a combined application (involving seed coat and foliar spray) of copper-chitosan NPs had enhanced the growth profile and crop productivity of finger millet plants. In addition, suppression of blast disease development via increased defense enzymes of finger millet plants by copper-chitosan NPs portrays the capability of NPs to enhance the disease resistance as extra merit to use in agricultural applications.
4.3. Effects of Carbon Based NMs
The CNMs are recognized as one of the most promising NMs in nanotechnology because of its intense applications. Thus, many phytotoxic studies have been performed to evaluate its potential toxicity.
4.3.1. Effects Of Multi Walled Carbon Nanotubes And Single Walled Carbon Nanotubes
Studies on red spinach, cucumber and lettuce showed that exposure of 1000 and 2000 mg/L concentrations of multi walled carbon nanotubes (MWCNTs) on hydroponic systems had significantly reduced the shoot and root elongation [48]. Zaytseva et al. [49] confirmed that phytotoxicity of MWCNTs coupled with the oxidative stress in relation to disturbance of micronutrient homeostasis. Shen et al. [50] experimented with single walled carbon nanotubes (SWCNTs) on protoplasts of Arabidopsis and Oryza and reported that SWCNTs have a dose-dependent programmed cell death through oxidative stress. Moreover, test plants had shown adverse cellular responses such as cell aggregation, chromatin condensation, and plasma membrane deposition.
4.3.2. Effects of Fullerene and Graphene NPs
Fullerene (C60) is another CNMs tested for phytotoxic effect in a study conducted by Santos et al. [51] on the aquatic plant Lemna gibba. They reported that the contents of chlorophylls a and b and the oxygen generation by chloroplasts were considerably decreased by the treatment of C60. Furthermore, graphene was evaluated for its phytotoxic effects on cabbage, tomato and red spinach at a concentration range of 500 to 2000 mg/L. Graphene caused an inhibitory effect on plant growth and biomass on test plants compared to their controls. In addition, the leaf number and the size of the leaves were considerably reduced in a dose-dependent manner. Moreover, concentration dependent intensification in reactive oxygen species generation, as well as cell death, was also observed along with necrotic lesions on test plants [52]. Hao et al. [53] suggested that exposure to 50 and 150 mg/L mesoporous CNMs negatively affect rice growth via altering the levels of vital phytohormones and also, toxicity was particle size-dependent. In addition, exposure to 150 mg/L mesoporous CNMs (150 nm) reduced root length and shoot lengths by 21% and 29%, respectively. Whereas 80 nm sized CNMs significantly reduced the root and shoot lengths by 70% and 57% at the concentration of 150 mg/L.
Despite their phytotoxic effects, some studies have emphasized the merits using CNMs on enhanced agricultural production. Morphologically different CNMs such as helical-MWCNTs, few-layered graphene, long MWCNTs, and short MWCNTs can activate cell growth, germination, and plant growth, in which tests performed on tobacco cell cultures confirms the growth was found to increase by 22%–46% when CNMs were introduced to the growth medium at a concentration of 50 μg/mL [54]. Application of graphene and MWCNTs on bioenergy crops (sorghum and switchgrass) increased the germination rate of switchgrass seeds and led to early germination of sorghum seeds. Furthermore, exposure of switchgrass to graphene (200 mg/l) enhanced the total biomass by 28% produced compared to untreated plants was reported by Pandey et al. [55]. Moreover, Pandey et al. [56] exposed MWCNTs and graphene had activated the early seed germination in Catharanthus and higher germination rate in cotton and Catharanthus seeds. Also, the soil growth of Catharanthus plants stimulated the reproductive system by inducing early flower development and accelerated total flower production by 37 and 58%, respectively.
Specifically, it was dictated that CNMs are multifaceted candidates to enhance plant growth as well as to eliminate the detrimental effects of environmental stresses from salt and drought with no symptoms on economically important crops [55, 56].
4.3.3. Effects of Carbon Dot NPs
Recently, Carbon dots (CDs) have been exploited as a novel form of CNMs showing a greater interest as markers for plant bioimaging. Because of their high aqueous solubility and flexibility in surface modification, the use of CDs has been spotlighted to be a promising substitute to conventional semiconductor quantum dots (QDs) and organic dyes [57-59]. When maize seedlings were exposed to 1000 and 2000 mg/L of CDs for 4 weeks, it was found that fresh weight of roots (57% and 68%) and shoot fresh weight (38% and 72%) were significantly reduced by CDs [60]. In contrast to other CNMs, the non-toxic carbon backbone of CDs shows much lower environmental toxicity and higher biocompatibility [58]. Li et al. [57] studied the phytotoxicity of fluorescent CDs on mung beans pointed out that, up to 0.4 mg/mL, there is a positive impact on the seed germination, root and stem elongation, fresh biomass, and moisture level of mung beans. Wang et al. [61] reported that CDs showed a dose-response effect on the growth of mung bean sprouts by promoting root elongation, stem elongation and biomass. In addition, mung bean sprouts treated with CDs had an elevated level in carbohydrate content by 21.9% compared to the control proving it a good candidate for fertilizers in agriculture.
4.4. Effects of Silica NPs
At an industrial scale, silica NPs rank top as a globally demanding product [14]. Silica NPs are widely utilized in fields like medicine, cosmetics, food industry, in biomedical and biotechnological applications as well as a nanofertilizer in agricultural applications [62]. Because of its extensive use, many studies have examined the potential toxicity of silica NPs on living beings [63]. Silicon (Si) is identified as a favorable element for the growth and development of plant systems where its contents are comparable to that of macronutrients essential for plant growth. Therefore, it has been widely used as a fertilizer to increase the yield of many crops [64]. Despite that, inherent physicochemical properties and the high reactivity of NPs led to assess the effects of silica NPs on seed germination and plant growth [14].
Silica NPs show phytotoxic effects principally at higher concentrations and sometimes the effects might depend on size, concentration and charge [65]. For example, at very high concentrations (540- 1820 mg/L), silica NPs had shown phytotoxic effects on Allium cepa with three different size ranges (7, 12, 22 nm). It was observed that silica NPs affected plant growth parameters such as germination and root elongation in seedlings. Moreover, cytogenetic analysis on root meristems has exhibited chromosomal abnormalities indicating its genotoxic effects [66]. The Bt- transgenic cotton plants tested with SiO2 NPs at 2000 mg/L showed a significant decrease in the plant height, shoot and root biomasses as well as affected the micronutrient contents such as Cu, Mg in roots and Na content in roots of transgenic cotton. In addition, the activity of superoxide dismutase and IAA content were considerably impacted by SiO2 NPs [67]. Therefore, it is important to improve the risk assessment of NPs under environmental exposures because the effects of NMs are concentration dependent as well as with the other physiochemical parameters.
Distinctive properties such as a large volume of tunable pores, high surface area, ease of surface functionalization, physical and chemical stability, high biocompatibility and low degradability under physiological conditions of mesoporous Si NPs, that make them ideal reservoirs for smart delivery systems [68-70]. Therefore, the applications of mesoporous Si NPs as a smart delivery system to increase the crop productivity has gained more attention in modern agriculture. Sun et al. [71] investigated the uptake of mesoporous SiNPs at specific concentrations (500 and 1000 mg/L) on wheat and lupin enhanced seed germination, plant biomass, leaf total protein and chlorophyll pigments. Also, the interaction of mesoporous SiNPs with the chloroplasts promotes plant growth by uplifting the photosynthesis of test plants.
4.5. Effects of Rare Earth Oxide NPs
Ma et al. [72] studied the effect of La2O3 NPs on the root growth of cucumber plants at concentrations over 200 mg/L. It was speculated that La2O3 NPs at the highest concentration (2000 mg/L) tested had shown a decrease in root elongation by 66% as a dose dependent response on cucumber. The release of Ce3+ ions plays a critical role in the phytotoxicity of CeO2 NPs on romaine lettuce was highlighted by Zhang et al. [73]. Romaine lettuce grown under sand containing CeO2 NPs had diminished the chlorophyll content by 16.5% and 25.8% at the highest concentrations tested (1000 and 2000 mg/kg). Moreover, there is a significant inhibition in the biomass production of lettuce as well as the concentrations assessed. Phytotoxicity of Y2O3 NPs on rice seedlings under hydroponic cultures was assessed by Zhao et al. [74]. Results indicated that high concentrations of Y2O3 NPs at 50 and 100 mg/L delayed seed germination. In contrast, lower concentrations including 1, 5, and 10 mg/L have shown positive effects on the root elongation of rice seedlings. Notably, Y2O3 NPs ranging from 20 to 100 mg/L significantly diminished the root activity and chlorophyll contents of rice. Furthermore, CeO2 NPs at 250 mg/L significantly reduced the disease severity to fusarium wilt while improving the chlorophyll content and the nutritional value of the tomato [75]. Therefore, all most all the studies available in the literature manifest the effect of different NMs have shown dose dependent responses to agricultural crops.
4.6. Effects of Polymeric and Other NPs
Chitosan/tripolyphosphate NPs had inhibited the germination Zea mays, Brassica rapa, and Pisum sativum. It was exploited that size, composition and the charge of the polymeric NPs had a significant effect with variable phytotoxicities [76]. Xin et al. [77] experimented on the effect of polysuccinimide polymeric NPs using Zea mays for germination and seedling growth with response to Cu stress. These NPs had improved the germination index of corn by mitigating the Cu stress. Also, it was reported as a novel opportunity to promote plant growth under heavy metal stresses. However, only a handful of studies have been performed on the effects of polymeric NPs on different model plants [76]. Tables 1 and 2 summarized the positive and negative effects of different NMs tested for phytotoxic effects. Madanayke et al. [78] and Kottegoda et al. [79] suggested hydroxyapatite NPs and their modified form with urea can be better macronutrient supplier enhancing crop growth.
Type of NMs | Size | Concentration | Test Plant | Effects | References |
---|---|---|---|---|---|
Superparamagnetic Fe-NPs | 9 nm | 0-2 mg/L | Soybeans | Increased the chlorophyll contents in sub-apical leaves | [24] |
ZnO NPs | 10 nm | 400 and 800 mg/kg | Cucumber | At 400 mg/kg ZnO NPs had significantly increased the starch content | [45] |
Green synthesized AuNPs | 30−113 nm | 5.4 ppm | Onion | Enhanced germination, plant height, leaf length, leaf diameter, neck diameter, and leaf surface area | [46] |
Helical-MWCNTs, layered graphene, long MWCNTs, and short MWCNTs | 13-18 nm | 50 μg/mL | Tobacco cell cultures | Activate cell growth, germination, and plant growth Cell growth was increased by 22%–46% when CNMs were introduced to the growth medium |
[54] |
Graphene and MWCNTs | 13–18 nm | 200 mg/l | Sorghum and switchgrass | Switchgrass to graphene enhanced the total biomass by 28% | [55] |
MWCNTs and graphene | 13-18 nm | Catharanthus and cotton |
Activated the early seed germination in Catharanthus and higher germination rate in cotton and Catharanthus seeds Stimulated the reproductive system by inducing early flower development and accelerated total flower production by 37 and 58% |
[56] | |
CDs | 4 - 6 nm | 0.02-0.12 mg/mL |
Mung bean | Promote root elongation, stem elongation and biomass Elevated levels in carbohydrate content by 21.9% |
[61] |
Mesoporous SiNPs | 20 nm | 500 and 1000 mg/L | Wheat and Lupin | Enhanced seed germination, plant biomass, leaf total protein and chlorophyll pigments | [71] |
CeO2 NPs | 8 ± 1 nm | 250 mg/L | Tomato | Reduced disease severity to fusarium wilt Improved chlorophyll content and the nutritional value |
[75] |
Type of NMs | Size | Concentration | Test Plant | Effects | References |
---|---|---|---|---|---|
CuO NPs | 30–50 nm | 10 g/L | Hordeum sativum | Inhibited growth by affecting the shoot and root elongations, maximal quantum yield of photosystem II, and transpiration rate | [36] |
CuO NPs in the presence of humic acid | 43 nm | 0, 2, 5, 10, 20, 50, 100 mg /L | Rice | Induced the inhibition of root elongation, abnormality in root morphology and its ultrastructure | [37] |
Fe2O3 NPs at concentration | 60 ± 27 × 30 ± 12 nm |
50 mg/L | Lactuca sativa | Decreased the root elongation by 20%, compared to control | [38] |
ZnO NPs | < 50 nm | 5 and 50 μg/mL | Allium cepa | Cytotoxic and genotoxic effects in the root meristems | [39] |
TiO2 NPs | 400 mg/L | Lactuca sativa | Negative impact on the nutritional quality | [40] | |
TiO2 NPs | 1000 ppm | Oryza sativa | Reduction in CO2 fixation, transpiration rate and stomatal conductance Effects on photosynthetic pigments, quantum efficiency of PSII (Fv/Fm ratio) and photochemical quenching |
[41] | |
TiO2 NPs | <25 nm | 0, 0.1, 1, 2.5, or 5% | Tobacco | Inhibit the germination rates, root lengths, and biomasses of tobacco seedlings | [42] |
Ag NPs | 20 nm | 50, 500, and 2000 mg/kg | Peanut | Reduction in plant growth and crop yield | [43] |
MWCNTs | Outer mean diameter ~13 nm, Inner mean diameter ~4 nm |
1000 and 2000 mg/L | Red spinach, cucumber and lettuce | Significantly reduced the shoot and root elongation | [48] |
SWCNTs | Diameter of 1 – 2 nm | 5–250 μ g/mL | Arabidopsis and Oryza | Dose-dependent programmed cell death through oxidative stress | [50] |
C60 | 29-38 nm | Lemna gibba | Contents of chlorophylls a and b and the oxygen generation by chloroplasts were considerably decreased | [51] | |
Graphene | 1 nm | 500 to 2000 mg/L | Cabbage, tomato and red spinach | Inhibitory effect on plant growth and biomass Leaf number and the size of the leaves were considerably reduced |
[52] |
Mesoporous CNMs | 80 nm and 150 nm |
50 and 150 mg/L | Rice | Exposure to 150 mg/L (150 nm) reduced root length and shoot lengths by 21% and 29%. Significantly reduced the root and shoot lengths by 70% and 57% at the concentration 150 mg/L (80 nm) |
[53] |
Silica NPs | 7, 12, 22 nm | 540- 1820 mg/L | Allium cepa | Affected on germination and root elongation in seedlings Cytogenetic analysis on root meristems showed chromosomal abnormalities |
[66] |
SiO2 NPs | 35 nm | 2000 mg/L | Bt- transgenic cotton | Decrease in the plant height, shoot and root biomasses Affected micronutrient contents such as Cu, Mg in roots and Na content in roots |
[67] |
La2O3 NPs | 65.8 ± 10.5 nm | 200 mg/L. - 2000 mg/L | Cucumber | Decrease in root elongation by 66% at the highest concentration | [72] |
CeO2 NPs | 16.5 ± 6.8 nm | 1000 and 2000 mg/kg | Lettuce | Diminished chlorophyll content by 16.5% and 25.8% at highest concentrations tested | [73] |
Y2O3 NPs | 20-30 nm | 50 and 100 mg/L | Rice seedlings | Delayed seed germination | [74] |
Hydroxyapatite NPs | diameter of 20 nm and an average length 150 nm | 0-10000 mg/L | Raphanus sativus | Hydroxyapatite NPs were biotransformed within the roots of the test plant. Shoot, root lengths and dry biomass were significantly enhanced with no effect on soluble protein and indole acetic acid content at the highest concentration tested. | [78] |
CONCLUSION AND FUTURE PROSPECTUS
Nanotechnology as an interdisciplinary approach has been linked with almost each and every field in science. Therefore, the utilization of NMs leads to release them deliberately or accidentally to the environment. Currently, agriculture has focused on using different nanoformulations on agricultural different applications, including fertilizers. However, NMs show differential effects of various plants based on their concentrations, environmental factors, and the tested species where it might either have positive or negative impacts. Despite their phytotoxic effects, NMs exhibits a range of beneficial effects with respect to the growth and development of plants that are particularly exposed to environmental stresses. Thus, it is important to rationally and safely apply NPs in agriculture as well as to establish a comprehensive system to effectively evaluate their impacts on crop plants. Therefore, in the future it important to exploit effective and safe strategies of application of different NPs incorporated products in agriculture. Also, more comprehensive studies should be carried out to evaluate the most effective concentrations of NPs on different crops as recommendations for agricultural applications. Finally, how to control their potential impact on food safety and food quality should draw more attention as NPs themselves could be taken up by crops and humans exposed to them through food consumption.
CONSENT FOR PUBLICATION
Not applicable.
FUNDING
None.
CONFLICT OF INTEREST
The author declares no conflict of interest, financial or otherwise.
ACKNOWLEDGEMENTS
The authors greatly acknowledge Isuru Priyaranga, teaching assistant, Department of Plant Sciences, University of Colombo for assistance with preparing the figure.