All published articles of this journal are available on ScienceDirect.
Present and Future Prospect of Algae: A Potential Candidate for Sustainable Pollution Mitigation
Abstract
Pollution control and mitigation are critical to protect the ecosystem and make everyone's life safer and healthier. Different pollution mitigation strategies and measures are implemented to remove pollutants, which broadly involve physical, chemical, and biological methods. Biological methods are found to be more sustainable, effective, and eco-friendlier than the other two methods. These methods mainly use microbes like bacteria, fungi, algae, and plants, and their products like enzymes and metabolic products to remove pollutants. Due to their unique photosynthetic ability and simple growth requirements, Algae can be grown using simpler components like CO2, sunlight, and media, making them a potential candidate to be used as a pollution mitigator. Algae can indicate and remove pollutants like CO2, SO2, NO2, and particulate matter from the air; these pollutants and particulate matter are either used for their growth or these are accumulated inside them.. Algal species have shown the efficient removal of heavy metals, organic pollutants, explosives, petroleum contaminants, pesticides, polycyclic aromatic hydrocarbons (PAHs), and plastics from different water sources. There is a lot of scope in using algae to remove organic and inorganic pollutants in wastewater treatment plants. Algae hold great potential to remove radioactive pollutants from natural resources and involve removal mechanisms like biosorption and bioaccumulation. Algae can be used with different adsorbent materials to develop adsorption systems for the adsorption of radionuclides and heavy metals. This review elucidates different algal species, their cultural conditions, the removal efficiency of different types of pollutants from the air, water, soil, and their role in genetic engineering and the algae's potential for waste mitigation.
1. INTRODUCTION
Due to the increase in population and rapid industrialisation, the pollution level in all the three-spheres is increasing day by day. Pollution in the atmosphere, hydrosphere, the lithosphere is disturbing the whole ecosystem and is causing serious harm to the creatures in the biosphere. Due to the air pollution in developing countries, serious health problems have arisen, causing loss of lives and a loss of money in the public health sector [1]. A rapid declination of forest and crop fields happens due to the deposition of air pollutants in acidic deposition, O3, SOx, NOx, and other oxidants [2]. Domestic wastewater, sanitary waste, agricultural wastewater, sewage, factory industrial waste, domestic waste disposal, insecticides, pesticides, fungicides, and chemical fertilisers, when mixed with natural water resources like a lake, pond, river, seas, and oceans, cause water pollution, and due to this, different water-borne diseases spread in human beings, as well as animals and the croplands and soil become infertile [3]. Due to the excessive use of fertilisers, insecticides, and pesticides, soil pollution takes place, which also, in turn, decreases soil quality and fertility. Apart from one of the major and probably most dangerous is pollution due to radioactive materials. The effluent of the nuclear power plant, uranium mining areas, medical and instrument manufacturing facilities, and nuclear weapon manufacturing facilities, when released into the natural environment, causes radioactive pollution. These radionuclides generally have a very long half-life and, due to this, they can accumulate in the bodies of humans and plants and cause cancer in human beings [4]. Sofia et al. provided detailed strategies for air pollution, which include short-term and long-term measures to be taken by citizens, enterprises, and public authorities [5]. Cooper et al. provided strategies to reduce and mitigate water pollution [6]. Different physical, chemical, and biological methods for mitigating radioactive waste have also been explained in a study [7]. When we consider biological methods for the treatment or removal of pollutants to mitigate pollution in all spheres, in most cases, it is found to be more efficient and environment friendly. Algae is a photosynthetic, autotrophic aquatic organism [8] that can be cultured and proliferated as it utilises CO2 and light energy (which are quickly and cheaply available) and can be used directly for the mitigation and removal of air, water, soil, and radioactive pollutants from the environment. The following review discusses the uses of algae as a candidate in different types of pollution mitigation.
2. AIR POLLUTION
Air pollution is currently a significant problem and needs serious attention. Air pollution is mainly driven by industrial activities, power plants, vehicle emissions; it contributes to about 80% of the overall pollution. The sources of air pollution are mainly divided into primary sources, natural sources, mobile sources, and indoor area sources [9]. According to WHO, air pollution caused the premature deaths of about 4.2 million people in 2016; by decreasing air pollution, we can decrease cardiovascular diseases, cancer, and other respiratory diseases [10]. Environmental Protection Agency (EPA), USA has set an ambient air quality standard for mainly six air pollutants, including particulate matter, lead, ground-level ozone, SO2, CO, and NO2 [11]. Hence, it is of the highest priority to develop strategies and solutions to decrease the concentration of such air pollutants to a safe limit. Air pollution can be mitigated by innovating new technology and developing alternative and more sustainable fuel sources [12]. Algae can be used to mitigate different air pollutants, hence they have great potential.
2.1. Pollution Indicator
Due to their unique nutrition requirement and habitat, algae are beneficial in detecting pollutants [13]. Any significant change in air quality can affect algal species. As algae generally use atmospheric air for their growth, any change in the air constituents can be sensed by algal species. Freystein et al. found a specific algal population growth where the particulate matter concentration is 10 [14]. There is significantly less algal population found in a high level of ozone concentration area, which suggests that the concentration of different air pollutants can relate to the occurrence of algal species.
2.2. Algal Mitigation
Algae, as photosynthetic organisms, can use CO2 as a carbon source and utilise it to produce different metabolites and sustain their growth cycle. Algae can use CO2 very efficiently to produce biomass, and the algal biomass can be used as different high-value products, hence they have an economic value [15]. Algal biomass and glucose can be used to develop a micropore adsorption system that provides a high surface area for CO2 capture [16]. Algae can be used in integrated systems to produce biofuels, treat wastewater, and it can be used for CO2 sequestration [17]. The process simulation of capturing CO2 by culturing algal biomass followed by oil extraction shows promising results [18]. Chlorella vulgaris grown in catholyte can sequester CO2 while sustaining power generation in Microbial Carbon Capture Cells (MCCs), implying that algae can be used for both carbon fixation and power generation in MCCs. [19]. Könst et al. explained the integrated production of different high-value products like antioxidants and essential fatty acids with the use of technology of CO2 capture by algae [20]. Alkaliphilic algal species like Phaeodactylum tricornutum, Chlamydomonas sp. show high levels of CO2 removal [21].
Algae can achieve high CO2 sequestration of about 80% to 99% at optimum conditions like pond water [22]. The use of different macro and microalgae that can be used to capture CO2 and for the generation of biofuels has been very extensively reviewed [23]. It is estimated that algal ponds sequester CO2 released from power plants efficiently and convert it as a carbon source for its growth [24]. It has been seen that a high concentration of CO2 and NO can be tolerated by Scenedesmus dimorphus and showed 75.61% CO2 utilisation efficiency when there is intermittent sparging of flue gas and pH feedback control system [25]. Algae can be screened based on their growth rate, photosynthetic capacity, environmental stress tolerance capacity, and ability to produce high-value products. It is found that Oscillatoria Blue-green algae can fix about 70 - 80% of the CO2 at optimal pH, light intensity, and temperature [26]. Chlorella vulgaris was cultured in an airlift photobioreactor and was 80% efficient for removing CO2 at the optimum temperature [27]. Spirulina sp. shows the highest biomass growth as the addition of CO2 increased up to 18% in vertical photobioreactor and can be effective for the removal of CO2 of higher concentration [28]. In all cases, CO2 is utilised in photosynthesis by algae and is considered the primary mechanism of CO2 sequestration. Spirulina sp. also sequester SO2 and NO2 and use them as a nutrient for their growth; they also have other potential uses [29]. Table 1 shows some of the algae and their air pollutant removal efficiency.
Algae species | Type of algae | Contaminant remediated | Efficiency | Reference |
Scenedesmus dimorphus | Green algae | CO2 | 75.61% | [25] |
Oscillatoria | Blue-green microalgae | CO2 | 70% - 80% | [26] |
Chlorella vulgaris | Green microalgae | CO2 | 80% | [27] |
Spirulina sp. | Blue-green algae | CO2 | Sustain 18% CO2 | [28] |
Spirulina | Blue-green algae | SO2, NO2 | - | [29] |
3. SOIL POLLUTION
Industrial solid waste and household waste disposal into the soil is a prevalent practice in this modern age. Soil contaminants primarily emerge from agriculture-related activities and waste from transportation industries. Many environmentalists visualise algae (microalgae in particular) as a potent tool for removing organic and inorganic pollutants in soil. Heavy metal pollution in soil emerges mainly from coal mines, copper mines, chromite mines, gold mines, electronic waste dumpsites, zinc smelting areas, and textile industries.
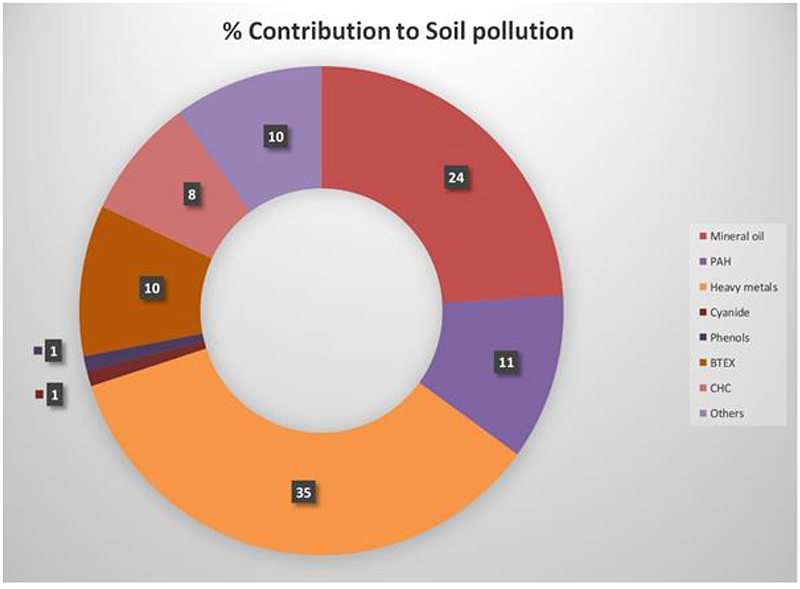
Effluent rich in heavy metal pollutants from these industries is leached down to the soil and then accumulated. Nickel, cobalt, chromium, and copper cause a high level of toxicity in plants, whereas mercury, arsenic, and lead cause toxicity in residing animals [30]. Significant contributors to soil pollution are shown in Fig. (1). Algal mitigation of different soil pollutants is elucidated.
3.1. Petroleum Contaminant
Petroleum hydrocarbons are the primary culprits of groundwater and soil pollution in industrial and urban areas. The significant impact it has is on organic carbon as 75% of carbon in oil is oxidisable, and it eventually causes a decrease in the pH of the soil [31]. The formation of a thick surface layer occurs when these hydrocarbons have contact with the soil, decreasing the exchange of gases between soil and air. This phenomenon leads to the suffocation of the plants, and hence the soil gradually becomes anaerobic, due to which soil microflora suffers a significant loss [32]. Walker and his coworkers showed the crude oil and motor oil-degrading capacity of alga Prototheca zopfii [33]. Phenols and sulfur-containing compounds are the major petrochemical contaminants. Biosorption and degradation of petroleum effluents by a cyanobacterium, Oscillatoria quadripunctulata, remove a significant 40% of TDS (Total Dissolved Solids) [34]. Navicula sp., a diatom, showed effective removal (> 50%) of 4-methyl cyclohexane acetic acid in two weeks [35]. Few algal species, such as Chlorella, Nostoc, and Ulva, are found to be capable of remediating petroleum-derived hydrocarbon under any O2 condition [36]. Table 2 shows different petroleum-associated contaminants removal by different algal species.
Algal biomass of Selenastrum capricornutum showed maximum efficiency in removing Benzo (a) pyrene compared to other pollutants. Biodegradation is the most effective mechanism in remediating associated petroleum contaminants.
3.2. Pesticides
Pesticides are being extensively tested with various algal species to establish a concrete relationship between pesticide concentration in water and its effect on the growth and metabolism of alga. Physical adsorption, biodegradation, complexation, and biotransformation are the few mechanisms identified as the true drivers of pesticide removal by the alga. Phycoremediation of a pesticide mixture with Atrazine as a principal constituent is being shown by freshwater algae Chlorella vulgaris and has a promising removal percentage of 98.6% to 98% [42]. Fluroxypyr is one of the most commonly used herbicides. Zhang et al. reported that the unicellular green alga Chlamydomonas reinhardtii removed this herbicide up to 57% through accumulation and degradation [43]. Table 3 shows the removal of pesticides by different algal species and the respective methods that have been adopted.
Algae species | Contaminant | Mechanism involved | Byproducts | The initial concentration of contaminants (mg/l) |
Removal efficiency (%) | Reference |
Chlorella vulgaris | Oil | Biodegradation or biosorption | - | 3 * | 41.56 | [37] |
Parachlorella kessleri LARG/1 | Benzene | Biodegradation | - | 0.1 | 40 | [38] |
Ethylbenzene | - | 30 | ||||
Toluene | - | 63 | ||||
Xylene | - | 40 | ||||
Agmenellum quadruplicatum PR-6 | Naphthalene | Biotransformation | 1Naphthol | 1.0 | 1.4 | [39] |
Selenastrum capricornutum | Benzo(a)pyrene | Biodegradation by dioxygenase | Diols of BaP | N.A. | 99 | [40] |
Chlamydomonas reinhardtii 11-32b | 9H-fluorene-9-one | Biodegradation | - | 6910 | 95.2 | [41] |
4-methylbenzo(c)cinnoline | - | 6760 | 4.3 |
Algae sp./Genus | Pesticide | Mechanism |
Initial concentration (mg/l) |
Removal efficiency (%) |
Reference |
Parachlorella kessleri | Bifenthrin | Biosorption and biodegradation | 100000 | 99 | [44] |
Chlorella vulgaris strain 211/11B | Mix M2101/1B | Biosorption | 0.0653 ± 0.0039 | 46.77 | [45, 46] |
Chlorella pyrenoidosa | Phenol and its derivatives | Biosorption and Biodegradation | 846 ± 2.2 | 90 | [47] |
Ankistrodesmus, Selenastrum | Atrazine | Biotransformation (Dealkylation) |
0.001 - 0.01 | N.A. | [48] |
Ankistrodesmus, Selenastrum | Fluometuron | Biotransformation (Dealkylation) |
0.005 | 75 | [48] |
Algae | PAH compound | Mechanism involved | Initial concentration (mg/L) |
Removal (%) | Reference |
Ulva prolifera | Naphthalene | Bio adsorption removal | 0.01 | 51 | [53] |
Phenanthrene | 0.01 | 79 | |||
Benzo[a] pyrene | 0.01 | 99 | |||
Selenastrum capricornutum | Fluoranthene | Bioaccumulation | 1.0 | 80.9 | [54] |
Oscillatoria sp. | Pyrene | Biodegradation | 200 | 95 | [55] |
Chlorella sp. | 78.7 | ||||
S. capricornutum, Chlorella sp. |
Benz[a]anthracene | Photodegradation | 100 | 47.4 | [56] |
Chlorella pyrenoidosa | Ceftazidime | Rapid biosorption followed by biodegradation | 100 | 92.70 | [57] |
After comparing various algal species in Table 3, it can be concluded that Bifenthrin is the best-remediated pesticide, and biosorption-biodegradation is the most efficient mechanism of remediation. In the case of remediation of phenol derivatives, the initial concentration beyond 60% (v/v) is a limiting factor as, beyond this concentration, the biosorption process is inhibited.
3.3. Polycyclic Aromatic Hydrocarbons (PAHs)
The US EPA labelled 28 PAH compounds as priority contaminants [49], and the majority of these arise due to incomplete combustion of agricultural remains. Soil concentration of PAH contaminant can reach as high as 300 g kg−1 [50]. PAH are listed amongst the hazardous materials because of their persistent nature. Chlorella sp.MM3 and Rhodococcus wratislaviensis strain 9 showed 100% removal of Phenanthrene, Pyrene, and BaP along with 13 other persistent PAH compounds from soil samples [51]. Selenastrum capricornutum, a green microalga, shows 88% of overall PHE (Phenanthrene) removal percentage in 96 h and 100% for FLA (fluoranthene) and PYR (pyrene) in 24 h [52]. Table 4 shows different algae species for PAH compound degradation.
Benzo [a] pyrene is the best remediated PAH compound with 99% removal efficiency. Adsorption is the most efficient removal mechanism followed by biodegradation. Chlorella sp. has shown the broadest applicability in terms of contaminant remediated.
3.4. Plastics
Polythene is a petroleum-derived product, and plastics are the organic polymers of the high-density polymer. Plastics are used in everyday life in many forms. Because of its ease of production and low cost, it is not commercially replicable. It is composed of toxic chemicals and non-biodegradable substances [58]. It is proven as a critical material for the growth of any developing nation. Its economic importance is evident from the fact that the annual production of plastic exceeds that of steel [59]. Adsorption of microplastics by green microalgae Skeletonema costatum and Chlamydomonas reinhardtii are found to be effective. The main advantage of using microalgae for microplastic treatment is its rapid physical adsorption [60, 61]. The percentage degradation of long density polythene by different microalgae [62] is shown in Fig. (2). Anabaena spiroides remediate Low-density polythene, and its ability to colonise in freshwater bodies and short doubling time makes it the best choice. The only limitation is the slow degradation even under laboratory conditions [62].
4. WATER POLLUTION
Urbanisation around the water bodies can be considered the primary culprit of 70% ground and surface water contamination. Natural sources of water pollution include open defecation by stray animals, an algal bloom in marine shores, volcanic eruption, and acid rains. Anthropogenic activities contributing to the deteriorating water quality include industrial effluent discharge, domestic sewage discharge, and oil spillage in marine oil refineries. Some of the significant contributors to surface and groundwater pollution are shown in Fig. (3).
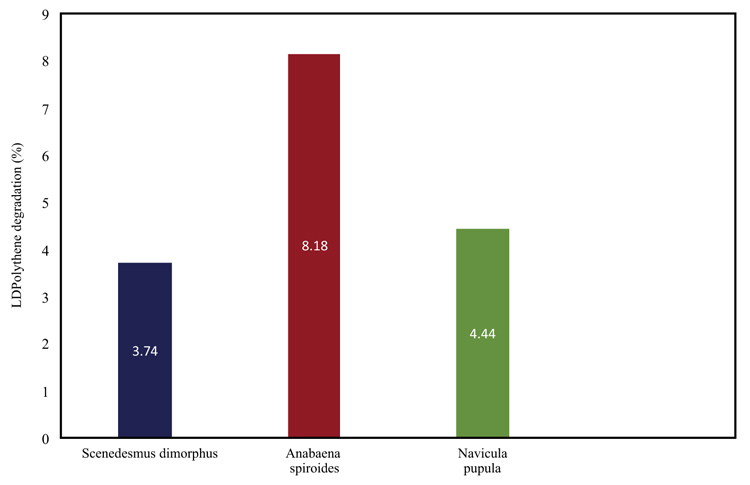
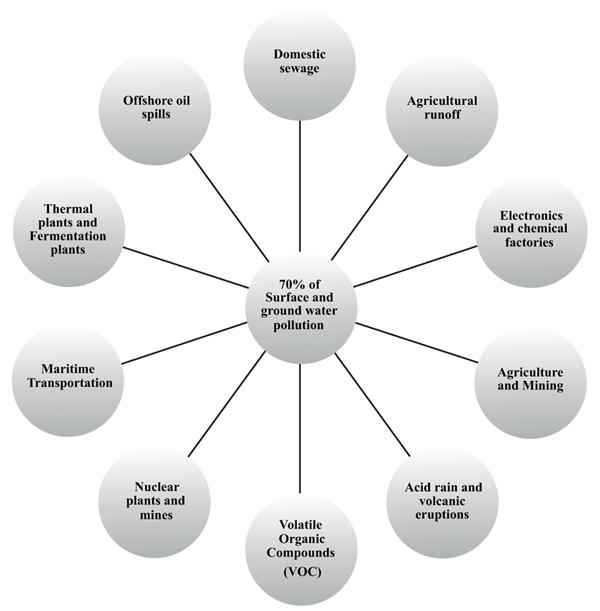
4.1. Heavy Metals
Industrialisation and urbanisation have worked hand in hand to gradually kill the remaining healthy water bodies near human settlements. Combustion of solid and wet waste, metallurgic activities, mining, maritime transportation, and extensive use of fertilisers to meet the food demand are a few of the causes of increased organic and heavy metal pollutants in the soil. Recently, researchers explored the potential of marine microalgae in the removal of heavy metal pollutants from water. Travieso et al. worked with two microalgae for demonstrating heavy metal removal. With the use of two different cell immobilisation methods, they did a comparative study. Predominantly, three heavy metals, cadmium, zinc, and chromium, weretargeted. With Kappa-carrageenan as an immobilisation method, the amount of heavy metal (Cd, Cr, and Zn) removal gradually increased compared to polyurethane foam in 24 h and 48 h period for both the algal species Chlorella vulgaris LAM-C30 and Scenedesmus acutus. However, in S. acutus, the difference in removal percentages for heavy metals of the two immobilisation methods is minute. C. vulgaris showed the average removal percentages of 61.5%, 81.5%, and 41% for Cd, Zn, and Cr, respectively. S. acutus showed a better removal percentage with 71%, 87.5%, and 33.5% for Cd, Zn, and Cr, respectively [63]. Table 5 shows heavy metals removal by various algal species along with their removal percentages.
By comparing the heavy removal efficiencies of various algal species, the study concluded that Zn is the best-remediated contaminant and Scenedesmus obliquus is the most efficient amongst them all. C. vulgaris is found to have the broadest range of applicability in bioremediation. Within a wide spectrum of mechanisms of heavy metal removal, biosorption is the most adopted one.
4.2. Organic Pollutants
Organic pollutants are of two types, biodegradable and Persistent Organic Pollutants (POPs). Tributyltin (TBT), Dimethyl Phthalate (DMP), p-nitrophenol, polychlorinated dibenzofurans, etc., are the few classes of POPs mainly contributing to the increasing amount of these compounds in the surface and groundwater reserves. Their persistent nature is due to the almost negligible or no solubility in water . The primary mechanism of action adopted by the majority of algal species is bioaccumulation, with few exceptions for bio adsorption and biosorption. Chlorella sp., Chlamydomonas sp., and Oscillatoria are the most promising organic pollutant removing agents [69]. The removal of bisphenol A by freshwater green algae Monoraphidium braunii with a maximum removal efficiency of 48% [70] turned out to be the early indicators of the hidden potential of microalgae in organic pollutant remediation. Selanastrum capricornatum and Cyanobacteria are used for the degradation of benzene, toluene, naphthalene, phenanthrene, pyrene in the contaminated water and soil [71]. Chlorinated hydrocarbons removal by 11 marine phytoplankton species [72] showed great potential for organic pollutant removal by freshwater microalgae as they have comparatively better accessibility and easy isolation.
4.3. Wastewater Treatment
Twenty-three metro cities contribute almost 60% of wastewater in India. Domestic sewage constitutes a large part of urban wastewater, and 80% of this wastewater is still discharged to water bodies untreated. It consists of lipids, fats, cellulose, heavy metals, several simple salts, and several pathogenic microbes. Biological Oxygen Demand (BOD) is the universally accepted norm for estimating contaminants in water bodies. Many blue-green algae, flagellated algae, and diatoms are predominantly residing in polluted rivers and drains. Hence the ability of several algal species to remove the water contaminants is extensively explored. Table 6 shows a list of algal species used in wastewater contaminant removal. Heavy metals contaminants discussed in section 4.1 hold a significant part in wastewater effluents from the industries. Manufacturing units and municipal facilities have toxic heavy metal pollutants in their effluent stream. An advantage of immobilised systems is that the biocatalyst and product may be separated easily, enabling effective product recovery (downstream processing). Another advantage is that the biocatalyst can be reused. The biological catalyst would be more stable in immobilised form.
Table 5.
Algae species | Heavy metal | Mechanism involved |
Initial Concentration (mg/l) |
Maximum removal % | Reference |
Closterium lunula | Cu | Bioaccumulation | 0.05 | 95 | [64] |
Chlorella pyrenoidosa | 79 | ||||
Scenedesmus obliquus | 67 | ||||
Desmodesmus pleiomorphus (strain L) | Cd | Biosorption | 0.5 | 98.8 | [65] |
C. pyrenoidosa | Zn | Biosorption | 5 - 10 | 95.6 | [66] |
Scenedesmus obliquus | 2.0 | 99.7 | |||
Oscillatoria agardhii BCC 52 |
Pb | Biosorption | 150 | 96 | [67] |
Lyngbya heironymusii BCC 41 | Cd | 200 | 97 | ||
Scenedesmus sp. BCC 82 | Hg | 600 | 97 | ||
Chlorella pyrenoidosa, C. vulgaris, algal–bacterial symbiosis (Chlorella + Nitzschia) |
Al | Biosorption | 25 - 63 | 65.4 | [68] |
Mn | 98.2 | ||||
Mg | 80 | ||||
Fe | 98.3 | ||||
Ca | 95.4 | ||||
Zn | 56.5 |
Algae species | Types of wastewater | Contaminant removal |
Removal percentage (%) |
Reference |
Chlamydomonas reinhardtii | Biomass Feedstock Production | N | 83 | [73] |
P | 14.45 | |||
Green algae mixture from a local Wastewater treatment plant | Dairy waste streams | NH4 | 96 | [74] |
Neochloris oleoabundans | Digested manure | N | 95 | [75] |
Planktothrix isothrix | Domestic wastewater | P | 100 | [76] |
Phosphorus-containing compounds were completely remediated from wastewater samples using algal biomass. Domestic sewage was efficiently remediated as compared to other effluent streams. Freshwater algae are efficient in remediating ammonia and have better applicability than any other algae.
4.4. Explosives
The most used explosive in the modern world is 2,4,6-trinitrotoluene (TNT). It is amongst the most significant marine pollutants due to numerous military installations and the testing of explosives-driven weapons along the seashore. TNT is relatively more soluble in water than other explosive chemical components [77]. Marine water pollutants are not well recorded and as significant as compared to other types of pollutants. TNT is highly toxic for the marine environment [78]. Three microalgae, Acrosiphonia coalita (green), Portieria hornemannii (red), and Pyropia yezoensis (red), are observed to have 100% TNT removal potential from seawater. 2-amino-4,6-dinitrotoluene and 4-amino-2,6-dinitrotoluene are the two leftover compounds of TNT degradation and constitute around 20% or less than the initial TNT amount. Hwang et al. worked with the microalga Scenedesmus obliquus to understand its biotransformation capabilities against hexahydro-1,3,5-trinity-1,3,5-triazine (RDX) [79]. The maximum transformation percentage was found to be 60%. Additional studies with green algae Pediastrum biwae showed up to 90% RDX transformation. Microalgae in the remediation of explosives have a considerable scope of research in the future, but currently, the literature is limited. A. coalita is considered the best choice for remediating TNT contaminants in water samples as it is distributed in a wide geographical area and can be easily found and isolated.
5. RADIONUCLIDE POLLUTION
Rapid industrialisation and an increase in population led to an increase in energy and other goods. Although the energy requirement can be fulfilled by generating energy using nuclear energy, due to its ability to produce and supply a large amount of energy as compared to other non-renewable sources like thermal energy, the radioactive waste produced from nuclear power plants, when mismanaged or released into the environment can damage the environment considerably. It is imperative to know the sources and the types of different radioactive materials to generate strategies for their removal. Radioactive wastes are mainly generated from the effluent of the nuclear power plant, uranium mining areas, medical and instrument manufacturing facilities, and nuclear weapon manufacturing facilities [4]. Radioactive waste can be classified based on half life (exempt waste, very short-lived waste, very low-level waste, low-level waste, intermediate-level waste, and high-level waste) or its physical state (aqueous radioactive waste, radioactive organic liquid, solid waste) [4, 80]. These radioactive wastes can harm the environment, organisms, plants, and humans directly or indirectly. When dumped into the soil or water sources, these radioactive nucleotides can accumulate over long periods and affect the soil microorganism and creatures, and aquatic animals. When they penetrate the plant, these radioactive wastes can cause genetic alteration in plants, which leads to the death of the plant. Radiation from radioactive waste can cause nausea, diarrhoea, vomiting on short term exposure and can cause permanent DNA damage and skin, lung, and thyroid cancers on long term exposure [80, 81].
5.1. Current Mitigation Strategies
Natarajan et al. critically reviewed the physical methods (incineration, distillation, evaporation, dumping), chemical methods (chemical precipitation, wet oxidation, acid digestion, immobilisation of radioactive waste), biological methods (microbial bioremediation, plant bioremediation, phytoextraction, phytovolatilisation, phytostabilisation, rhizofiltration, phytodegradation) for the treatment of radioactive waste [80].
5.2. Algal Removal of Radionuclides
Algae can remove radionuclides from the environment by the biosorption or bioaccumulation method. Algae can also be used with other composite materials to form adsorbent material for the adsorption of radioactive materials. Some of the algae are reported to have resistance to high ionising radiations like α-rays, making them a potential candidate for removing radionuclides. Algae use different survival techniques to withstand the high ionising radiation (active DNA repairing) and use different mechanisms (ion channels, complex formation, binding of specific domains) to uptake radioactive nucleotides.
Krejci et al. reported that Closterium moniliferum, a freshwater Charophyceae of desmid order having a crescent shape, can bioaccumulate the strontium from the water and store it in its subcellular location, i.e., in the vacuole in crystal form. Strontium, due to its similarity of atomic size and properties with calcium, is very dangerous especially, Sr-90 (a radioisotope of strontium) due to its long half-life (30 years) and can enter into the human body and cause cancer. Closterium moniliferum accumulates barium and calcium and co-accumulates the strontium due to its structural similarity with calcium and eventually precipitates to form crystals. Hence, by exploiting this property, Sr-90 accumulation can be increased [82, 83]. Rivasseau et al. isolated Coccomyxa actinabiotis (CCAP 216-25), an extremophile unicellular freshwater eukaryotic green microalga, which can tolerate massive ionising radiation doses with a value of about 20 000 Gy by possibly utilising its specific resistance and repair mechanisms. They also found that C. actinabiotis can uptake and accumulate different types of radionuclides, having 100% removal efficiency of Ag-110, Zn-65, Cs-137, and up to 95% uptake in the case of U-238. It also shows an uptake rate of about 90 - 91% in the case of Co-60, Co-58, and Mn-54 [84, 85]. Jha et al. found and evaluated free-floating algal species' ability to accumulate Ra-226 in water bodies near radioactive mining areas. Chara sp., Nitella sp. are filamentous algae, and aquatic plants like Pistia sp., Jussia sp., Eichornia sp., Hydrilla shows a high level of Ra-226 accumulation. The activity concentration ratio of Ra-226 in filamentous algae and aquatic plants to the activity concentration in water shows excellent value, which varies from 1.1×103 up to 8.6 ×104 [86]. Zalewska and Saniewski studied the bioaccumulation activity of Polysiphonia fucoides and Furcellaria lumbricalis, and Rhodophyta found in the Baltic and found that Polysiphonia fucoides show greater bioaccumulation of Cr-51, Zn-65, Ag-110m, Sn-113, Cs-137, and Am-241. Mn-54, Co-57, Co-60 show greater activity concentration in Furcellaria lumbricalis, and hence both of these show promising features for the bioaccumulation of radionuclides [87]. Veliscek Carolan et al. reported that during a dose assessment study, Ulva sp. and Ecklonia radiata that grew near a sewage treatment plant showed concentration factors 176 and 526 bioaccumulation of I-131 [88]. Radiation tolerance study with the cyanobacterium Chroococcidiopsis reported that these algae could survive x-rays of radiation up to the value of 15 kGy as compared to the extremophilic bacterium Deinococcus radiodurans which shows the highest ionising radiation survival rate [89, 90]. The mechanism adopted by Chroococcidiopsis is that it can repair the damaged DNA very fast and efficiently, which also happens during the DNA damage when there is dehydration [91].
It has been reported that along with the indicator of trace elements, red alga Jania longifurca found and isolated from the coast of Syria shows the bioaccumulation of Pb-210 (26.01 ± 2.60 Bq kg-1) and J. longifurca shows the bioaccumulation of Po-210 (27.43 ± 0.58 Bq kg-1). Brown algae like Cystoseira sp. and P. pavonia show the bioaccumulation activity for Po-210 (26.40 ± 1.10 Bq kg-1 and 24.43 ± 0.66 Bq kg-1, respectively), but Cs-137 accumulation was less in all cases [92]. Farhi et al. performed ionising-radiation tolerance studies on green micro-alga Chlorophyceae followed by Spectroscopic investigation and found that alga can tolerate high γ -irradiation up to 6 kGy. Spectroscopic investigations show the release of amino acids, which shows that the probability of intense DNA repair activity was due to radiation [93]. Bioaccumulation studies of Cs+ and Cs-137 by nine strains of algae were conducted and found that Desmodesmus armatus can remove Cs+ by bioaccumulation. The process of bioaccumulation is followed by a separation process where magnetic nanoparticles coated with polyethyleneimine are used for the separation of the algae, which has accumulated Cs-137. Adding organic substrates could improve Cs+ uptake by algae. On the other hand, if K+ ion concentration is more than 10 mg/l, it will competitively decrease the rate of Cs+ absorption by algae, possibly because both the ions use the same transport channel for the transportation of ions across the boundary of the cell. It is also reported that the bioaccumulation activity is high at higher pH (8-9) and also increases with an increase in initial Cs concentration [94]. Alissa et al. reported that Spirogyra, which is a filamentous charophyte, can accumulate uranium with a concentration factor of a maximum of 67 and have an average concentration factor of about 22. Cladophora sp., which is a filamentous Ulvophyceae, can accumulate uranium with a concentration factor of a maximum of 280 and have an average concentration factor of about 250 [95]. It is found that Ulva sp. and Na bentonite composite shows biosorption/adsorption of uranium ions from aqueous solutions with a high adsorption rate under optimum pH, temperature, and initial uranium concentration, etc [96]. Lee et al. reported that Haematococcus pluvialis red cyst shows a high value of biosorption and removal of almost 95% radioactive Cs-137 in 48 h, which is higher than Chlorella vulgaris and Anabaena sp. The mechanism involved for Cs-137 accumulation involves uptake through a potassium transport channel, binding with caesium binding domain on the red cyst surface, and complex formation with a functional group [97]. Khani et al. studied the biosorption of uranium by Cystoseria indica in a batch system. It is reported that C. indica shows maximum absorption with optimum temperature and pH conditions. Absorption increases with an increase in initial uranium concentration with an adsorption value in the range of 198 mg/g to 233 mg/g [98]. A freshwater Chlorophyta, Graesiella emersonii isolated from hot spring, shows maximum sorption capacities of Ra-226 and U-238 under optimum pH and initial cell concentration, which affects the biosorption system considerably and hence plays an essential role in scaling up the process [99].
It is found that the biosorption of uranium by non-living biomass of the Sargassum fluitans, a Phaeophyceae, after protonation shows bioaccumulation of uranyl ion from aqueous solution at optimum pH. High uranium adsorption at high pH (4.0) happens due to the presence of hydrolysed uranyl ions [100]. It is reported that Padina sp., a brown alga is a potential species that shows biosorption of uranium. The algae show an adsorption capacity of 434.8 mg/g at 10°C with a defined uranium concentration [101]. Bampaiti et al. reported that a brown alga Dictyopteris polypodioides shows biosorption of U (VI) ions in batch technique under optimum conditions; they also reported that biosorption under defined experimental conditions like pH, initial uranium concentration, biomass concentration, temperature show accumulation efficiency up to 94% [102]. Decontamination study of removal of low-level Co-60 and Cs-137 reported that Haematococcus sp. (freshwater species of Chlorophyta) showed 62.3% removal efficiency for low-level Co-60 concentration in 2 days and also showed the removal of Cs-137 with an efficiency of 88% in 48 hrs [103]. Extremophilic Arthrospira platensis, a filamentous cyanobacterium, shows the removal of radionuclides by the biosorption and bioaccumulation process. A. platensis showed high bioaccumulation and biosorption capacity for Pu-239, Sr-90, and Am-241 and showed an increased uptake of U-233 when polyphosphates were added to the cultivation medium [104, 105]. Dabbagh et al. reported that Oscillatoria homogenea filamentous cyanobacterium could remove Sr-90 and stable strontium from the aqueous solution at an optimum pH after ten days of incubation. Biovolume of cyanobacteria, illumination, initial Sr concentration, and incubation times shows a considerable effect on the bioaccumulation and biosorption of Sr [106]. Table 7 shows a list of different algal strains, radioactive contamination list, and mechanisms involved in the removal.
Fukuda et al. performed a global search for the potential algal and plant strains to determine their ability to bioaccumulate accidentally released radionuclides from the nuclear power plant in Fukushima, which involves the radionuclides like Cs-137, Sr-90, as these radionuclides due to their analogy with potassium and calcium, respectively can accumulate inside a living organism, for example, accumulation of Sr-90 in the bone. They found that out of 188 strains of algae and plant strains, some algae strain shows a very good efficiency in the accumulation of Sr-90, Cs-137, and I-125. Stigonema ocellatum, Oedogonium sp., Egeria densa show the bioaccumulation of Sr-90 with an efficiency of 41.3%, 36.3%, and 33.9%, respectively. Algal species like Nostoc commune, Scytonema javanicum, Stigonema ocellatum, Ophiocytium sp. show the bioaccumulation of I-125 with an efficiency of 65.9%, 61.9%, 48.5%, and 41.6%, respectively. A freshwater Eustigmatophyceae strain nak-9 which was later identified as Vacuoliviride crystalliferum shows up to 90% bioaccumulation of Cs-137 in 1 hr. Other than that, Batrachospermum virgato-decaisneanum, Chloroidium saccharophilum also shows the bioaccumulation of Cs-137 with an efficiency of 37.9% 22.4%, respectively. Stigonema ocellatum shows the elimination of both Sr-85 and I-125. Lemna aoukikusa shows the elimination of both Cs-137 and I-125, respectively [107-109]. Another extremophilic unicellular red alga (Rhodophyta), Galdieria sulphuraria, shows the bioaccumulation of Cs-137 from a medium deprived of potassium-containing caesium concentration of 30 µg/l with a removal efficiency of 52 ± 15% in mixotrophic growth condition with a culture period of 10 days [110]. Data's efficiency of different algae strains is shown in the table. Table 8 shows the bioaccumulation efficiency of different algae, their type, mechanism of removal, and the concentration of radionuclides.
Algae Species (Strain) | Contaminant remediated |
Mechanism involved in removal |
Reference |
Coccomyxa actinabiotis (CCAP 216-25) |
Ag-110, Co-60, Co-58, Sb-124, Cr-51, Zn-65, Mn-54, Cs-137, U-238, C-14 | Bioaccumulation | [84, 85] |
Chara sp., Nitella sp. | Ra-226 | Bioaccumulation | [86] |
Closterium moniliferum | Sr-90 | Bioaccumulation in crystal form | [82, 83] |
Polysiphonia fucoides, Furcellaria lumbricalis | Cr-51, Zn-65, Ag-110m, Sn-113, Cs-137, Mn-54, Co-57, Co-60 | Bioaccumulation | [87] |
Ulva sp., Ecklonia radiata | I-131 | Bioaccumulation | [88] |
Cyanobacterium Chroococcidiopsis |
X rays (up to 15 kGy) | Ionizing-Radiation Resistance | [91] |
Jania longifurca, Cystoseira sp., P. pavoni | Pb-210, Po-210, Cs-137 | Bioaccumulation | [92] |
Chlorophyceae | high γ -irradiation (up to 6 kGy) | Ionizing-Radiation Resistance | [93] |
Desmodesmus armatus SCK | Cs-137 | Bioaccumulation | [94] |
Spirogyra, Cladophora spp. | Uranium | Bioaccumulation | [95] |
Ulva sp.and sodium bentonite composite | Uranium ions | Adsorption | [96] |
Haematococcus pluvialis CCAP 34/7 red cyst | Cs-137 | Biosorption and Bioaccumulation | [97] |
Cystoseira indica | Uranium | Biosorption | [98] |
Graesiella emersonii (Shihira and R.W. Krauss) |
Ra-226 | Biosorption | [99] |
Sargassum fluitans | Uranium | Biosorption | [100] |
Padina sp. | Uranium | Biosorption | [101] |
Dictyopteris polypodioides | U (VI) ions | Biosorption | [102] |
Haematococcus sp. | Low-level Co-60 and Cs-137 | Bioaccumulation | [103] |
Arthrospira platensis CNMN–CB-02 | Pu-239, Sr-90, Am-241, U-233 | Biosorption and Bioaccumulation | [104], [105] |
Oscillatoria homogenea | Sr-90 | Bioaccumulation and biosorption | [106] |
Algae species (Strain) | Type of Algae | Contaminant remediated | Initial concentration (µg ml-1) | Efficiency (%) | Reference |
Stigonema ocellatum (NIES-2131) | Freshwater cyanophyceae | Sr-85 | 0.0071 | 41.3 | [107] |
Oedogonium sp. (nak 1001*) | Freshwater Chlorophyceae | 0.0071 | 36.3 | ||
Egeria densa (We2*) | Freshwater Magnoliopsida | 0.0071 | 33.9 | ||
Nostoc commune (TIR 4*) | Terrestrial Cyanophyceae | I-125 | 5.9 | 65.9 | |
Scytonema javanicum (NIES-1956) | Terrestrial Cyanophyceae | 5.9 | 61.9 | ||
Stigonema ocellatum (NIES-2131) | Freshwater Cyanophyceae | 5.9 | 48.5 | ||
Ophiocytium sp. (nak 8) | Freshwater Xanthophyceae | 5.9 | 41.6 | ||
Batrachospermum virgato-decaisneanum (NIES-1458) |
Freshwater Florideophyceae | Cs-137 | 0.0022 | 37.9 | |
Chloroidium Saccharophilum (NIES-2352) |
Freshwater Chlorophyta | 0.0022 | 22.4 | ||
Vacuoliviride crystalliferum (nak 9) | Freshwater Eustigmatophyceae | 0.0022 | 60 (15 min) - 90 (within 1 h) | [107], [108] | |
Galdieria sulphuraria 074 W (NIES-3638) | Extremophilic unicellular red alga (Rhodophyta) | 0.03 | 52 ± 15 | [110] |
Algae species (Strain) | Chemical modification | Contaminant remediated | Initial concentration (ng/10 ml) | Efficacy (%) |
Nostoc corneum | Non-phosphorylated dry alga mass | 85-Sr 226-Ra 241-Am |
23 14 2.3 |
72.2 91.7 99.4 |
Nostoc corneum | Phosphorylated dry alga mass | 134-Cs 85-Sr 226-Ra |
1 23 14 |
79.3 97.8 98.6 |
Nostoc insulare (Borzi 54.79) |
Non-phosphorylated dry alga mass | 85-Sr 226-Ra 241-Am |
23 14 2.3 |
74.8 87.8 85.7 |
Oscillatoria geminata (Meneghini B 1459-8) |
Non-phosphorylated | 85-Sr 226-Ra 241-Am |
23 14 2.3 |
90.4 96.1 96.7 |
Oscillatoria geminata (Meneghini B 1459-8) |
Phosphorylated dry alga mass | 134-Cs 85-Sr 226-Ra |
1 23 14 |
78.0 85.5 87.6 |
Spirulina laxissima (G.S. West B 256.80) |
Non-phosphorylated dry alga mass | 85-Sr 226-Ra 241-Am |
23 14 2.3 |
92.5 93.8 69.4 |
Cs-134, Am-214, Sr-85, Ra-226, can be adsorbed by cyanobacteria biomass. Nostoc corneum, Nostoc insulare, Oscillatoria geminate, Spirulina laxissima, and waste product from alginate production, which contain algal species like Laminaria digitata and Laminaria japonica, have shown different uptake efficiency for different radionuclides. It is also reported that phosphorylated cyanobacterial biomass shows improved efficiency of radionuclide adsorption [111]. Some of the removal efficiency of phosphorylated and non-phosphorylated cyanobacterial strain was shown in Table 9.
Most of the studies suggest that algae found in extreme habitats show a higher ability to remove radionuclides from the environment. However, their limitation involves providing optimum conditions to grow the algae so that they can show maximum efficiency to remove the contaminants. Algae can be a potential candidate for the mitigation of radioactive wastes. Bioaccumulation and biosorption of radionuclides by algae highly depend on types of culture operation, pH, media constituents, contact time, initial biomass load, initial radionuclide concentration, light intensity, and adsorbent material. Hence, further research in the above areas and isolation and screening of new algae can be the potential area of research.
6. GENETIC ENGINEERING APPROACHES
Engineering different wild-type algal strains using genetic engineering tools and understanding the biochemical pathways of algae can have a significant impact on improving algae's ability to remove pollutants more efficiently. Chlamydomonas and Chlorella are two of the most successful models for studying different genetic engineering techniques [112]. Fayyaz et al. provided a detailed review of the use of different advanced genetic engineering tools to improve the biorefinery capacities of algae [113]. Chen et al. engineered Synechococcus elongatus a cyanobacterium to produce carbonic anhydrase, which can convert CO2 to HCO3- and HCO3- is found to have a positive effect on algal growth as algae can use HCO3- as a carbon source for photosynthesis and more biomass yield and thus enhances the CO2 capture efficiency. The results show the capture of 441.8 mg of CO2 per litre of genetically engineered biomass higher than the wild type, which captures 331.8 mg per litre of biomass [114]. Carbon fixation phenomena are directly proportional to the photosynthetic efficiency of microalgae species. Beckmann et al. showed that by transforming the NAB1, an RNA-binding protein that is present in the cytosol of Chlamydomonas reinhardtii mutant strain and plays an essential role in regulating (translation repression) the size of the light-harvesting antenna (LHC), would increase the photosynthetic efficiency by 50% under high light intensity. This increased photosynthetic capacity will undoubtedly result in higher carbon fixation rates than parent strain [115]. Algae's photosynthetic efficiency and CO2 capture ability can be improved considerably by modifying its light-harvesting complexes using genetic engineering methods [116]. The photo-inhibition of microalgae at high light intensities decreases the algae's photosynthetic ability, consequently lowering CO2 capture efficiency. CRISPR/ cas9 systems and genetic engineering tools have been used to modify Photosystem II, and LHCII antennas have shown improved photo-protection capacity [117]. Sakshi and Ak provided a detailed review of different PAH degrading catabolic enzymes and the application of genetic engineering for improved PAH degradation. Engineering different algae with PAH-catabolic enzymes can improve the efficiency of algae to degrade PAH [118]. Algae can be engineered to express metal-binding proteins on its surface, which can adsorb Hg and Cd by binding onto metallothionein [119, 120]. Huang et al. expressed mercuric reductase in Chlorella sp. by engineering algae with the MerA gene isolated from Bacillus megaterium algae produces mercuric reductase, which converts Hg2+ to volatile Hg and shows higher growth rate and photosynthetic activity [121]. CrMTP4 gene, a cation diffusion facilitator/membrane transporter when overexpressed in C. reinhardtii, increases both the tolerance and uptake rate of Cd metals [122]. AtHMA4, a heavy metal ATPase found in Arabidopsis thaliana as a transgene and its C-terminal domain when transferred into C. reinhardtii shows increased metal tolerance ability and the bioaccumulation Cd and Zn [123]. An increase in lindane (organic pesticide) degradation was noticed in Nostoc ellipsosporum transformed with the linA gene [124]. Genetically modified Anabaena sp., possessing Pseudomonas paucimobilis gene that controls the first step of lindane catabolism, degraded the parent compound 1,2,4-trichlorobenzene [125]. The use of genetic engineering tools to increase the efficiency of microalgae for the removal of pesticides is not yet explored and has potential research areas for the future [126].
CONCLUSION
Algae show great potential for the removal of pollutants. The efficiency of removal of different pollutants depends mainly on the type of algal strain, location from where it is isolated, culture conditions which involves optimum pH, temperature, light intensity, media composition, trace element concentration in media, initial pollutant load, initial biomass load, the contact time of the pollutant with algae. In some cases, cultural mode (photoautotrophic, photoheterotrophic, heterotrophic, mixotrophic) also affects the efficiency of removal of pollutants. Algae isolated from extreme conditions have shown potential for the accumulation of heavy metals and radioactive materials. Algae can remove the pollutants by direct uptake or involve mechanisms like biosorption and bioaccumulation. Dry algal biomass shows the potentiality to be used as adsorbent material for the adsorption of pollutants. These types of algae also show high resistance to ambient changes. Future research may involve isolation of more potential algal strains, development of more optimised culture conditions for efficient removal of pollutants, genetic engineering of the wild strains to improve pollutant removal efficiency. Considering the increase in pollution rate, scaling up pollution mitigation while maintaining efficient removal by algae is the need of the hour.
CONSENT FOR PUBLICATION
Not applicable.
FUNDING
None.
CONFLICT OF INTEREST
The authors declare no conflicts of interest, financial or otherwise.
ACKNOWLEDGEMENTS
The authors are highly grateful to the management of Lovely Professional University for providing necessary research facilities.