All published articles of this journal are available on ScienceDirect.
Silver Nanoparticles Affect the Inflammatory Response in a Lung Epithelial Cell Line
Abstract
Background and Objectives:
Silver nanoparticles (AgNPs) have a dual effect showing both inflammatory and anti-inflammatory effects; however, the molecular mechanism of their anti-inflammatory effect is not clearly understood. In this study, we investigated the effect of AgNPs on the inflammatory response.
Methods:
We induced an inflammatory response in a lung epithelial cell line using tumor necrosis factor-α (TNFα) as an in vitro inflammatory model. Then the effect of AgNPs on the TNFα-induced inflammatory response was observed.
Results:
The mRNA expression of pro-inflammatory cytokines (IL-1β and IL-18) showed upregulation of IL-1β by AgNPs alone. However, AgNPs reduced the TNFα-induced upregulation of IL-1β and IL-18. AgNPs reduced the TNFα-induced NF-KB response, reactive oxygen species (ROS) generation, Nod Like Receptor Family-Pyrin domain containing 3 (NLRP3) gene expression, and caspase-1 activation, indicating that the anti-inflammatory effect of AgNPs was by inhibition of both NF-KB transcriptional and inflammasome pathways. Conversely, AgNPs alone induced the activation of both NF-KB transcriptional and inflammasome pathways, suggesting their involvement in the molecular mechanism of the inflammatory effect of AgNPs.
Conclusion:
Altogether, these findings show that two different pathways are involved in the molecular mechanism of both the dose-dependent inflammatory effect of AgNPs alone and the anti-inflammatory effect of AgNPs against the TNFα-induced inflammatory response. Understanding this mechanism will help to improve the medical applications of AgNPs and suggest their potential as a TNFα inhibitor to treat TNFα-induced inflammatory diseases.
1. INTRODUCTION
Nanotechnology is an emerging field with significant medical potential. Silver nanoparticles (AgNPs) are widely used in many consumer products ranging from textiles to deodorant sprays and many medical applications [1-3]. AgNPs have a dual effect; many in vitro studies have reported their cytotoxic effect in different cell lines [4, 5], yet their protective anti-microbial and anti-inflammatory effects are widely known [6, 7]. However, the exact mechanism of the anti-inflammatory effect of AgNPs is not fully understood. Clarification of the AgNPs-induced anti-inflammatory mechanism is important to improve their medical applications.
To investigate the anti-inflammatory effect of AgNPs and its mechanism, an appropriate inflammatory in vitro model should be used. Tumor necrosis factor-α (TNFα) is a pro-inflammatory cytokine that regulates cellular responses in many pathological conditions such as inflammatory diseases and cancer [8]. Inflammation is a major cellular response of TNFα and is mainly induced by the NF-KB activation pathway [9]. Therefore, the TNFα-induced inflammatory response in lung epithelial cells is a suitable model to study the mechanism of the anti-inflammatory effects of AgNPs.
In our previous research, we investigated the effect of AgNPs on TNFα-induced apoptosis and DNA damage responses and concluded that the expression of tumor necrosis factor receptor 1 (TNFR1) on the cell membrane is reduced by AgNPs, resulting in reduced signal transduction of TNFα, including its apoptotic and DNA damage effect [10, 11]. Due to the potential of AgNPs to interfere with the cellular responses of TNFα, we hypothesized that AgNPs might also affect the inflammatory cellular response by their effect on TNFR1. Therefore, both the NF-KB and inflammasome pathways were investigated to help clarify the molecular mechanism of the AgNP effect.
Inflammasomes are multiprotein complexes that activate the inflammatory response [12]. Inflammasome activation results in the activation of caspase-1 and secretion of the pro-inflammatory cytokines IL-1β and Il-18 [13]. Inflammasome activation is commonly induced by a wide range of nanofibers and nanotubes such as silica, asbestos, and carbon nanotubes and can drive lung inflammation and fibrosis [14, 15]. Recently, the activation of inflammasomes by particulate nanomaterials has been investigated, and it was found that titanium dioxide and silica nanoparticles induce inflammasome activation in different cell lines [16, 17]. Moreover, AgNPs can induce inflammasome activation in human hepatoma cell lines in a size-dependent manner [18]. Therefore, examining the inflammasome pathway can clarify both the inflammatory and anti-inflammatory effects of AgNPs, along with assessing the NF-KB pathway.
In this study, we investigated the dose-dependent effect of AgNPs on the TNFα-induced inflammatory response in lung epithelial cells, along with the molecular mechanism. We used TNFα to induce the cellular inflammatory response, then the response after exposure to AgNPs to clarify the mechanism of the anti-inflammatory effect was investigated. We also investigated the response of the lung epithelial cell line to the direct effect of AgNPs alone without induction of inflammation by TNFα. Our results revealed that both inflammatory and anti-inflammatory effects of AgNPs could be induced by activation or inhibition of both the NF-KB transcriptional and inflammasome pathways. These findings will help improve the use of AgNPs in medical applications.
2. MATERIALS AND METHODS
2.1. Cell Line and Culture
The human pulmonary epithelial cell line NCI-H292 (ATCC CRL-1848TM) was used in this study. To culture the cells, RPMI-1640 medium (L-glutamine with phenol red, Nacalai Tesque, Japan) supplemented with 10% (v/v) heat-inactivated fetal bovine serum (HFBS, Biowest, USA), 100 μg/ml penicillin, and 10 μg/ml streptomycin (Nacalai Tesque) was used. Cells were incubated at 37°C in a humidified atmosphere containing 5% CO2.
2.2. Silver Nanoparticles (AgNPs)
Polyvinylpyrrolidone (PVP)-coated AgNPs were purchased from Sigma-Aldrich (Cat. No. 576832) with a particle size of less than 100 nm. The mean size was approximately 50-90 nm as measured by transmission electron microscopy (TEM; JEM-2000FX, JEOL, Japan). Electronic light scattering (Zeta-potential & Particle Size Analyzer ELSZ-2000, Otsuka Electronics, Japan) measurements showed that the hydrodynamic diameter of AgNPs was 170 ± 50 nm, the polydispersity index was 0.20, and the zeta potential was -53.2 mV. AgNPs were spherical in shape with slight aggregations in deionized water.
2.3. Tumor Necrosis Factor-α (TNFα)
Recombinant human TNFα (Peprotech, USA) was reconstituted in water to 100 µg/ml. The required working concentrations were diluted in sterilized culture medium (RPMI-1640) containing a carrier protein.
2.4. Gene Expression Analysis by Real-time (RT) PCR
For mRNA expression analysis of interleukin 1 beta (IL-1β), interleukin 18 (IL-18), and Nod Like Receptor Family-Pyrin domain containing 3 (NLRP3), cells were seeded at a concentration of 4 × 105 cells/60 mm cell culture dish. Cells were then exposed to AgNPs (10 and 100 µg/ml) and/or TNFα (20 ng/ml) for 8 h. After that, cells were detached, collected, and the total cellular RNA was extracted using an RNeasy kit (Qiagen, USA) according to the manufacturer’s protocol. An aliquot (1 µg) of the extracted total RNA was reverse transcribed into cDNA using a RT2 First Strand kit (SABiosciences/Qiagen, USA). The PCR primers for human IL-1β, IL-18, and NLRP3 were purchased from SABiosciences/Qiagen. The reaction mixture was prepared following the manufacturer’s protocol. Glyceraldehyde-3-phosphate dehydrogenase (GAPDH) was used as a house-keeping gene to normalize the data. RT-PCR analysis was performed using an ABI PRISM 7000 sequence detection system (Applied Biosystems, Singapore), and the thermocycling conditions were 95°C for 10 min, followed by 40 cycles of 95°C for 15 s and 60°C for 1 min.
2.5. Dual-luciferase Reporter Assay for NF-KB Response Assessment
2.5.1. Plasmids
pGL3-Control vector (E1741, Promega) was used as an empty control reporter plasmid. NF-KB promoter-reporter plasmid (E8491, Promega) was employed to detect the inflammation. Both reporter plasmids contain SV40 promoters and enhancer sequences that result in strong expression of the luciferase encoding gene (luc+) in different types of mammalian cells. Also, pRL-CMV vector (E2261, Promega), which is a Renilla luciferase-encoding control plasmid, was used as an internal control to determine the transfection efficiency.
2.5.2. Transfection
pGL3 blank control reporter plasmid or NF-KB promoter-reporter plasmid and pRL-CMV internal control plasmid were co-transfected into NCI-H292 cells. LipofectamineTM LTX reagent with a PlusTM reagent kit (Invitrogen) was used to perform the transfection according to the manufacturer’s protocol. Cells were cultured at a density of 2 × 105/ml in a 24-well plate and incubated overnight at 37°C. Opti-MEM medium (Life Technologies, USA) was used to dilute the Lipofectamine LTX reagent and plasmids, then the Plus reagent was added to the diluted plasmids. Diluted plasmids with Plus reagent were added to the diluted Lipofectamine LTX reagent at a 1:1 ratio and incubated for 5 min at room temperature. Finally, the plasmid-lipid complexes were added to the cells and incubated at 37°C for at least 24 h before exposure to TNFα and AgNPs.
2.5.3. Assessment of Luciferase Activity
After exposure of transfected cells to TNFα (20 ng/ml) and AgNPs (10 or 100 µg/ml) separately and together for 12 h, the luciferase activities were assessed using a Dual-Luciferase Reporter Assay System (E1910, Promega) according to the manufacturer’s protocol. Cells were lysed using 1× passive lysis buffer and gentle shaking for 10 min, then cell lysates were transferred to tubes containing luciferase assay reagent II (LAR II). Firefly luciferase (F) signals were measured, then Stop & Glo reagent was added to the tubes, and the Renilla luciferase (R) signals were measured as well. The firefly and Renilla luciferase signals were recorded using a luminometer (Lumat LB9507, BERTHOLD TECHNOLOGIES, Germany) according to the instrument manual. The changes in luciferase activities were calculated using the following equation:
![]() |
2.6. DCF Assay for Oxidative Stress Determination
To quantify intracellular reactive oxygen species (ROS), a ROS assay kit (OxiSelectTM, Cell Biolabs, Inc., USA) was used. This assay is based on the cell permeable fluorogenic probe 2’,7’-dichlorodihydrofluorescein diacetate (DCFH-DA), diffuses into cells is deacetylated by intracellular esterases to the nonfluorescent 2’,7’-dichlorodihydrofluorescein (DCFH). In the presence of ROS, DCFH is rapidly oxidized to the highly fluorescent 2’,7’-dichlorodihydrofluorescein (DCF). The fluorescence intensity is proportional to the intracellular ROS levels.
Cells were cultured at a density of 1 × 104 cells/well in a black 96-well plate and incubated overnight. Subsequently, media were removed, and cells were washed twice gently with DPBS (14190, GIBCO, Invitrogen, USA) and incubated with DCFH-DA/media solution for 30 min in the dark at 37°C. Then, after removing the solution and washing the cells with DPBS, the DCFH-DA-loaded cells were exposed to TNFα (20 ng/ml) and AgNPs (10 or 100 µg/ml) separately and together for 12 h. Parallel sets of wells containing DCFH-DA-loaded cells without any further exposure were used as a negative control. The fluorescence of DCF was measured at an excitation/emission wavelength of 480 nm/530 nm using a fluorometric plate reader (Microplate Fluorometer, Twinkle LB 970, BERTHOLD TECHNOLOGIES). The amounts of produced DCF were calculated based on a DCF standard curve.
2.7. Caspase-1 Activity Assay
To monitor the activity of caspase-1, a caspase-1/ICE fluorometric assay kit (Biovision, USA) was used. This assay is based on the detection of the cleavage of the substrate YVAD-AFC, which emits blue light; after its cleavage by caspase-1, free AFC emits a yellow green fluorescence. According to the manufacturer’s protocol, cells were exposed to TNFα (20 ng/ml) and AgNPs (10 or 100 µg/ml) separately and together. After 12 h of exposure, cells were pelleted, resuspended in cell lysis buffer, and incubated on ice for 10 min. Then, 2× reaction buffer and YVAD-AFC substrate were added to the cell lysate and incubated at 37°C for 1-2 h. Fluorescence was then measured using a fluorometric plate reader (Microplate Fluorometer, Twinkle LB 970, BERTHOLD TECHNOLOGIES) at an excitation/emission wavelength of 400 nm/505 nm. The fold increase in caspase-1 activity was determined by comparing the activity of the enzyme in the samples with the activity of the untreated cells.
2.8. Immunostaining and Confocal Laser Scanning Microscopy
To analyze the expression and localization of purinergic 2X7 receptor (P2X7R), NCI-H292 cells were seeded in a CELLview cell culture dish (Greiner Bio-one North America Inc., USA) at a density of 1.5 × 104 cells/compartment and incubated for 24 h. The cells were exposed to TNFα (20 ng/ml) only, or together with AgNPs (10 or 100 µg/ml). After 12 h of exposure, the cells were washed with 1× PBS fixed with 4% formaldehyde solution in PBS (Wako) at room temperature, permeabilized with 0.1% Triton X-100, and then blocked with 10% normal goat serum in PBS for 1 h. The cells were then incubated overnight at 4°C with rabbit polyclonal anti-P2X7 receptor antibody (Alomone, Israel) followed by incubation with labeled goat anti-rabbit IgG H&L (Alexa Fluor 594) (Ab150080, Abcam) for 1 h at room temperature. Nuclear DNA was stained with DAPI (4′, 6-diamidino-2-phenylindole) (Dojindo, Japan) for 5 min at room temperature. The stained samples were mounted by antifade solution (Fluoromount, Diagnostic Biosystems, Canada). Microscopic observations and images were acquired using a confocal laser-scanning microscope (Leica TCS SP5, Wetzlar, Germany) with a 63 × 1.4 Plan-apochromat oil immersion lens.
2.9. Statistical Analysis
Statistical analysis was performed using Student’s t test. Differences and significances between means of different groups were determined using one-way ANOVA with Duncan’s multiple comparison tests. *P < 0.05, **P < 0.01, ***P < 0.001 were considered statistically different as shown in figures. Data are presented as means ± standard deviation (SD) with at least three independent replicates (n ≥ 3).
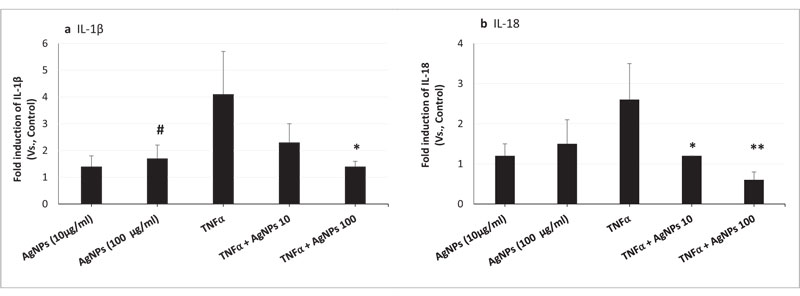
3. RESULTS
3.1. Effect of AgNPs on TNFα-induced pro-inflammatory Cytokines Secretion
IL-1β and IL-18 are pro-inflammatory cytokines and belong to the IL-1 superfamily. Both are important mediators of the inflammatory cellular response, including fever generation, secretion of other cytokines and chemokines, and attraction of immune cells [19, 20]. TNFα can induce the secretion of IL-1β [21] and IL-18 [22] in different cell lines. Because of the known anti-inflammatory effect of AgNPs, we investigated the effect of AgNPs on TNFα-induced IL-1β and IL-18 secretion in NCI-H292 cells.
We conducted a gene expression assay to analyze the IL-1β and IL-18 mRNA expression levels. As shown in Figs. (1A and B), TNFα-induced IL-1β and IL-18 gene expressions were significantly reduced by the exposure of cells to both TNFα and AgNPs (100 µg/ml), from 4.1- to 1.4-, and from 2.6- to 0.6-fold, respectively. Also, the reduction in the expression of both genes was dose-dependent; increasing the concentration of AgNPs from 10 to 100 µg/ml resulted in greater reduction in TNFα-induced IL-1β and IL-18 secretions. These results demonstrated the suppression of TNFα-induced Il-1β and Il-18 mRNA expression levels by AgNPs, suggesting they have an anti-inflammatory effect.
On the other hand, AgNPs alone induced IL-1β mRNA expression in NCI-H292 cells as shown in Fig. (1A). This result indicated the induction of pro-inflammatory cytokine expression, showing that AgNPs have an inflammatory effect.
3.2. Effect of AgNPs on the TNFα-induced NF-KB Response
Secretion of pro-inflammatory cytokines is regulated at the transcriptional and post-transcriptional levels. The signal transduction of TNFα and its receptor (TNFR1) is the first signal that might be involved in the secretion of active pro-inflammatory cytokines (IL-1β and IL-18) via the NF-KB transcription pathway [23]. Therefore, we transfected an NF-KB promoter-reporter plasmid to detect the inflammatory response and to check whether the NF-KB pathway is involved in the effect of AgNPs on the TNFα-induced IL-1β and IL-18 secretion.
As shown in Fig. (2), the NF-KB response in cells exposed to AgNPs alone was dose-dependent. However, when cells were exposed to both TNFα and AgNPs (100 µg/ml), the fold induction of the NF-KB response was significantly reduced from 2.4 to 1 compared to the TNFα-exposed cells. These results revealed suppression of the TNFα-induced NF-KB response by AgNPs, suggesting that the NF-KB pathway is involved in the anti-inflammatory effect of AgNPs against the TNFα-induced inflammatory response. Also, by activation of the NF-KB pathway, AgNPs can induce their inflammatory effect in a dose-dependent manner.
3.3. Effect of AgNPs on the TNFα-activated Inflammasome Pathway
The second signal involved in the secretion of active pro-inflammatory cytokines (IL-1β and IL-18) is the activation of caspase-1. Caspase-1 cleaves pro-IL-1β and pro-IL-18 into their biologically active forms, IL-1β and IL-18 [24]. Caspase-1 activation can be initiated by NLRP3 inflammasome activation [25], and the generation of ROS can induce NLRP3 inflammasome activation [26]. TNFα has been reported to mediate this inflammasome pathway in a human neuroblastoma cell line [21]. Therefore, we investigated the inflammasome pathway to assess its role in the effect of AgNPs on the TNFα-induced inflammatory response.
ROS generation, NLRP3 mRNA expression, and caspase-1 activity were increased in a dose-dependent manner in cells exposed to AgNPs (10 and 100 µg/ml) alone as shown in Figs. (3, 4, and 5) respectively. On the other hand, the cells exposed to both TNFα and AgNPs together showed a dose-dependent significant decrease in both TNFα-induced ROS generation and NLRP3 mRNA expression as shown in Figs. (3 and 4). However, the TNFα-induced caspase-1 activity was significantly reduced only by the higher concentration (100 µg/ml) of AgNPs as shown in Fig. (5). These results suggested that the inflammasome pathway is involved in the molecular mechanism of the anti-inflammatory effect of AgNPs against the TNFα-induced inflammatory response. Moreover, AgNPs induced inflammasome activation in a dose-dependent manner, suggesting the dual activities.
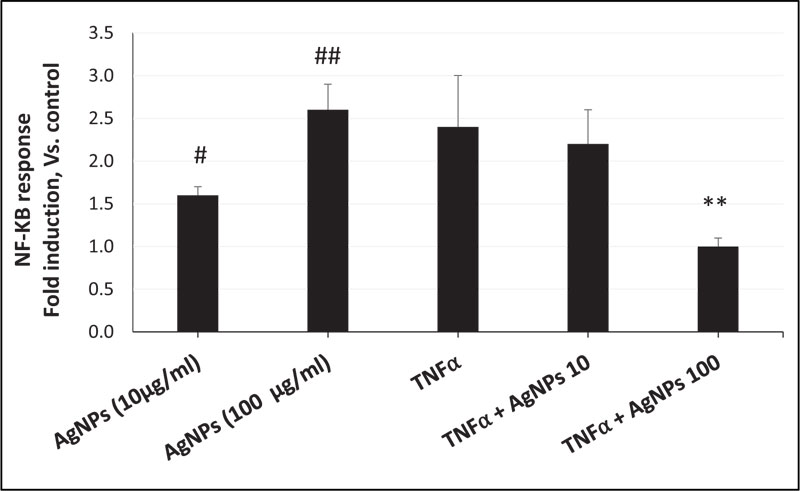
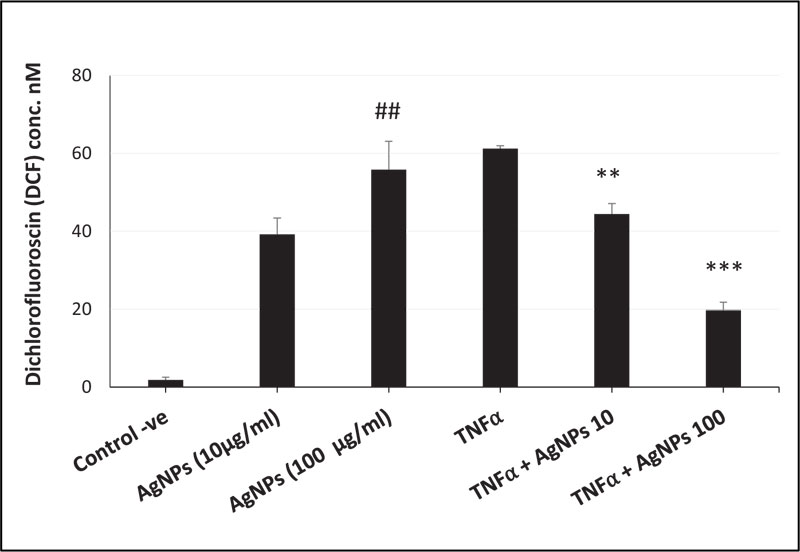
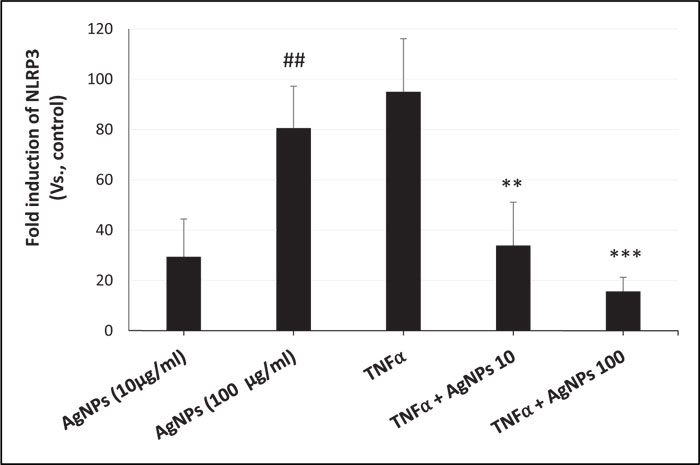
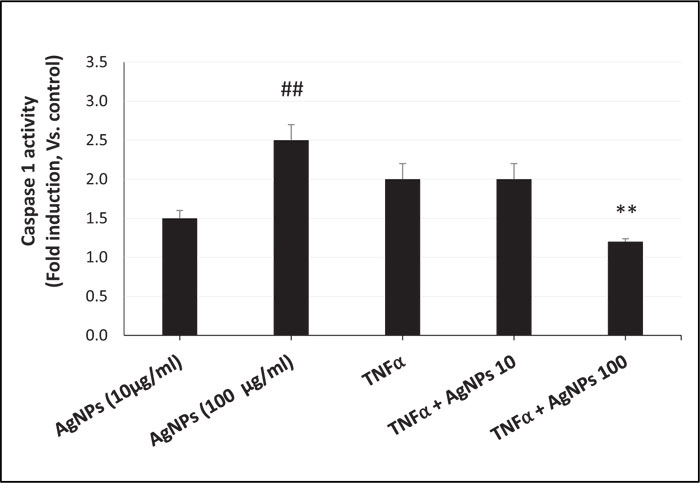
3.4. Expression of Purinergic 2X7 Receptor (P2X7R)
P2X7R is a ligand-gated ion channel that is activated by extracellular ATP [27]. The activation of P2X7R plays an important role in the inflammatory response, including ROS generation [28, 29], NLRP3 inflammasome activation [30, 31], and release of pro-inflammatory cytokines such as IL-1β and IL-18 [32]. Moreover, P2X7R has been implicated in lung inflammation induced by TiO2 and SiO2 nanoparticles via inflammasome activation and IL-1 secretion in lung epithelial and macrophage cell lines [33]. Due to the impact of P2X7R, we hypothesized that the interference of AgNPs with the inflammatory response of TNFα might be affected by P2X7R activation. Therefore, we quantified the expression of P2X7R using immunofluorescence assays and confocal microscopy. As shown in Fig. (6A-D), P2X7 receptors were expressed in NCI-H292 cells after exposure to TNFα alone and to TNFα + AgNPs (10 and 100 µg/ml). Cells exposed to TNFα + AgNPs (100 µg/ml) showed a significant decrease in the expression of P2X7 receptors compared to cells exposed to TNFα alone and cells exposed to TNFα + AgNPs (10 µg/ml).
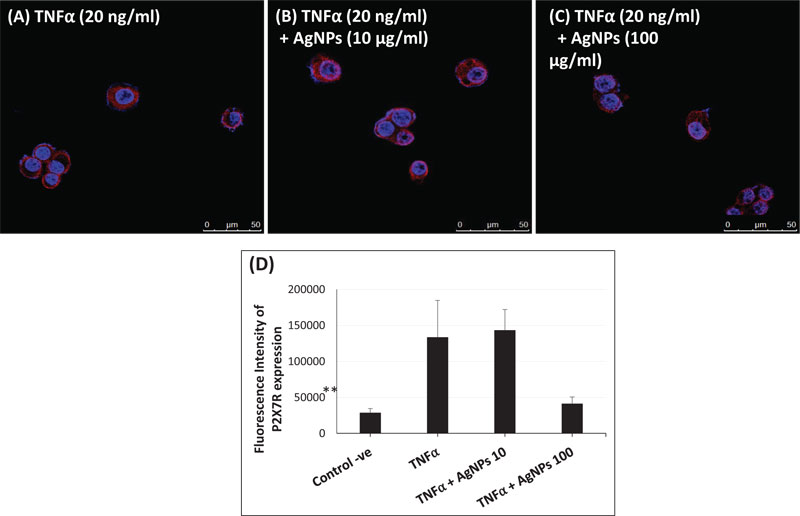
These data suggest that AgNPs, together with TNFα, reduced the expression level of P2X7R on the cell membrane, and this resulted in inhibition of P2X7R signal transduction, resulting in reduced TNFα-induced pro-inflammatory cytokine release. This suggests that inhibition of the TNFα-induced P2X7R/NLRP3 inflammasome pathway by AgNPs is a possible molecular mechanism of their anti-inflammatory effect.
4. DISCUSSION
AgNPs are widely known for their antimicrobial effect, which had led to their use in many medical applications such as deodorant sprays, wound dressings, and catheter coatings [34, 35]. However, AgNPs can induce many different cellular responses, such as cell proliferation, inflammation, genotoxicity, and cell death [36-38]. Different cellular responses of AgNPs are affected by the different characteristics of AgNPs themselves, such as concentration, size, coating, and surface charge. For example, AgNPs with a size of 3-5 nm can induce inflammation in a neural cell line [39], while synthetized AgNPs with size range of 40-100 nm enhance the anti-inflammatory effect in human peripheral blood mononuclear cells [40], showing the double-edged effect of AgNPs. Therefore, AgNPs should be characterized well to specify the induced cellular response. In this research, we conducted a dose-dependent study of negatively charged, PVP-coated AgNPs with a mean size of 50-90 nm. PVP-coated AgNPs are recommended for in vitro studies because of their higher stability with less aggregation and dissolution changes as compared to other coatings, such as citrate and polyethylene glycol (PEG) [41].
Because of the widespread medical applications of AgNPs, we hypothesized that the response of healthy cells would be different than that of cells in diseased conditions such as bacterial infection, sepsis, cancer, or inflammatory disease. Therefore, we designed our study to assess the effect of AgNPs on lung epithelial cells in both healthy and diseased cell conditions. For this purpose, we used TNFα as an inflammatory-inducing agent and lung epithelial cells to create an in vitro inflammatory disease model. Then we investigated the effect of AgNPs on lung epithelial cells with and without TNFα. TNFα is known to have autocrine activity, suggesting endogenous TNFα also could induce pro-inflammatory cytokines [42].
The release of pro-inflammatory cytokines (IL-1β and IL-18) as a mediator of the inflammatory response was analyzed in this study. The results of both IL-1β and IL-18 mRNA expressions showed a dose-dependent reduction in the TNFα-induced mRNA expressions, revealing an anti-inflammatory effect for AgNPs. However, AgNPs (10 and 100 µg/ml) induced an inflammatory response in non-treated cells by inducing pro-inflammatory cytokine expression as shown in Fig. (1A, B). In order to understand the molecular mechanism of the change in TNFα-induced pro-inflammatory cytokines released by AgNPs, we assumed that the NF-KB transcriptional pathway would play a role in this effect. The NF-KB transcriptional pathway is a known signal transducer of TNFα, and is stimulated by the binding of TNFα to its receptor TNFR1. Our results revealed the suppression of the TNFα-induced NF-KB response by AgNPs, especially the higher concentration (100 µg/ml), as shown in Fig. (2). Moreover, AgNPs alone induced the NF-KB response in a dose-dependent manner, confirming the involvement of the NF-KB transcriptional pathway in both inflammatory and anti-inflammatory effects of AgNPs. We could relate this change in the NF-KB transcriptional pathway to the change in the expression of TNFR1 on the cell membrane. Specifically, we have concluded that the AgNPs can reduce the expression of TNFR1, resulting in a reduction in TNFα-induced signal transduction in a lung epithelial cell line [10]. So, this AgNP effect induced a reduction in the activation of the TNFR1/NF-KB transcriptional pathway, resulting in a reduction in the TNFα-induced inflammatory response as shown in Fig. (7).
The inflammasome activation pathway in this study, in addition to the NF-KB transcriptional pathway, to assess its involvement in both the inflammatory and anti-inflammatory effects of AgNPs was investigated. Inflammasomes are complexes consisting of NLRP3, apoptosis-associated speck-like protein (ASC), and pro-caspase 1 [43]. Many stimuli can activate inflammasomes such as pathogen-associated molecular patterns (PAMPs) [44], environmental toxins [45], and lipopolysaccharides (LPS) [46]. Nanoparticles can also induce inflammasome activation [47]. The generation of mitochondrial ROS by different stimuli leads to NLRP3 inflammasome activation [48], resulting in caspase-1 activation. Caspase-1 then converts pro-interleukins (pro-IL-1β and pro-IL-18) to active forms (IL-1β and IL-18) and initiates the inflammatory response. In this study, we investigated the ROS-mediated inflammasome pathway. Our results showed that ROS generation, NLRP3 mRNA expression, and caspase-1 activity were increased in a dose-dependent manner in non-treated cells exposed only to AgNPs (10 and 100 µg/ml) as shown in Figs. (3, 4, and 5) respectively. This result shows how AgNPs could activate the inflammasome pathway by ROS generation and then NLRP3 mRNA expression and caspase-1 activation, which lead to pro-inflammatory cytokine release, explaining the inflammatory effect of AgNPs by themselves. On the other hand, the inflammatory cell model (TNFα-induced inflammatory response) showed significant dose-dependent decreases in TNFα-induced ROS generation, NLRP3 mRNA expression, and caspase-1 activity after exposure to AgNPs (10 and 100 µg/ml), revealing their anti-inflammatory effect against the TNFα-induced inflammatory response. These findings indicate that AgNPs can activate and inhibit the ROS-mediated inflammasome pathway, inducing inflammatory and anti-inflammatory effects respectively depending on the condition of cells (with or without TNFα) as shown in Fig. (7).
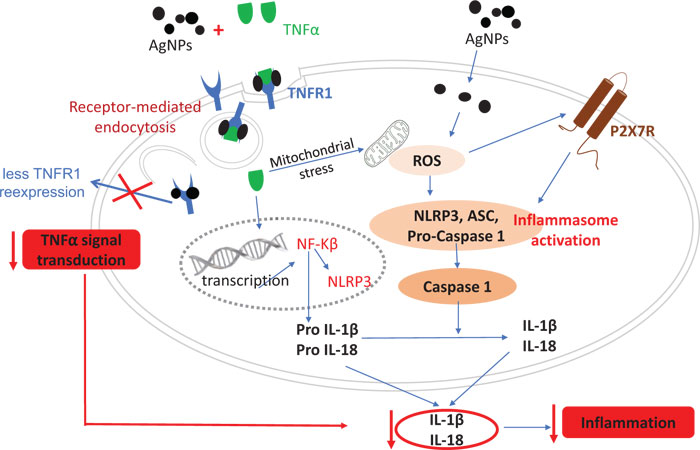
Since Purinergic 2X7 receptor (P2X7R) plays a key role in the inflammation process, including NLRP3 inflammasome activation [49, 50], we hypothesized that the mechanism of the AgNPs-induced anti-inflammatory effect might be mediated through the expression of P2X7R. P2X7R is activated by extracellular adenosine triphosphate (ATP) and then induces the passage of Ca2+, Na+, and K+ ions across the plasma membrane [51]. Activation of P2X7R induces the generation of ROS [52] and subsequently will induce inflammasome activation and the inflammatory response. The expression of P2X7R was quantified in this study using immunofluorescence assays and confocal microscopy as shown in Fig. (6). The high concentration of AgNPs (100 µg/ml) induced a significant decrease in the TNFα-induced expression of P2X7R compared to the low concentration of AgNPs (10 µg/ml). This result indicates that AgNPs can reduce extracellular ATP production, which then reduces the P2X7R activation. So, we can relate the anti-inflammatory effect of AgNPs to reduced P2X7R expression, which reduces ROS generation, NLRP3 inflammasome activation, caspase-1 activation, and pro-inflammatory cytokines (IL-1β and IL-18) release; all of these events would finally reduce the inflammatory response as shown in Fig. (7).
Finally, our findings provide details about the possible molecular mechanism of both the inflammatory and anti-inflammatory effects of AgNPs. This molecular mechanism occurs by two different pathways. The first pathway is the NF-KB transcriptional pathway, which is regulated by TNFα/TNFR1 signal transduction, which is inhibited by AgNPs resulting in a reduction in pro-inflammatory cytokine (IL-1β and IL-18) release, eventually reducing the TNFα–induced inflammation in the in vitro disease model. However, this pathway can be activated by AgNPs alone, producing an inflammatory effect in non-treated cells. The second pathway is the Inflammasome pathway, which is regulated by both ROS generation and the expression of P2X7R. In the in vitro disease model, the inflammasome pathway would be inhibited by AgNPs via a reduction in ROS generation or P2X7R expression, and then a reduction in subsequent events including the NLRP3 inflammasome activation, caspase-1 activation, and pro-inflammatory cytokines (IL-1β and IL-18) release, eventually reducing TNFα-induced inflammation. However, in non-treated cells, AgNPs could activate the inflammasome pathway, producing an inflammatory effect. In this study, we elucidated some of the details of the anti-inflammatory effect of AgNPs to help to improve their medical applications. Moreover, our findings suggest that AgNPs can be used as a TNFα inhibitor to treat TNFα-induced diseases such as Alzheimer’s disease. To further explore the therapeutic potential of AgNPs, in vivo studies are needed to complement in vitro results of this study.
CONCLUSION
In this study, we found a dose-dependent anti-inflammatory effect of AgNPs against the TNFα-induced inflammatory response and proposed a molecular mechanism for this effect. This mechanism works by inhibition of either the NF-KB transcriptional pathway or the inflammasome pathway, resulting in reduced pro-inflammatory cytokines (IL-1β and IL-18) release, eventually reducing TNFα-induced inflammation. Moreover, AgNPs alone induced the inflammatory response in healthy cells by activation of both pathways, revealing that AgNPs are a double-edged sword depending on their concentration and cellular conditions.
ETHICS APPROVAL AND CONSENT TO PARTICIPATE
Not applicable.
HUMAN AND ANIMAL RIGHTS
No human or animals were used in this research.
CONSENT FOR PUBLICATION
Not applicable.
AVAILABILITY OF DATA AND MATERIALS
Not applicable.
FUNDING
None.
CONFLICT OF INTEREST
The authors declare no conflict of interest, financial or otherwise.
ACKNOWLEDGEMENTS
We thank Ms. Hiromi Morita and Mr. Shinya Hattori of the NIMS Molecule & Material Synthesis Platform for assistance in obtaining and analyzing the flow cytometry results.