All published articles of this journal are available on ScienceDirect.
Bioremediation of Heavy Crude Oil Contamination
Abstract
Crude oil contamination is one of the major environmental concerns and it has drawn interest from researchers and industries. Heavy oils contain 24-64% saturates and aromatics, 14-39% resins and 11-45% asphaltene. Resins and asphaltenes mainly consist of naphthenic aromatic hydrocarbons with alicyclic chains which are the hardest to degrade. Crude oil biodegradation process, with its minimal energy need and environmentally friendly approach, presents an opportunity for bioremediation and as well for enhanced oil recovery to utilize heavy oil resources in an efficient manner. Biodegradation entails crude oil utilization as a carbon source for microorganisms that in turn change the physical properties of heavy crude oil by oxidizing aromatic rings, chelating metals and severing internal bonds/chains between molecules. Biodegradation does not necessarily lower quality of crude oil as there are cases where quality was improved. This paper provides information on heavy crude oil chemistry, bioremediation concept, biodegradation enzymes, cases of Microbial Enhanced heavy crude Oil Recovery (MEOR) and screening criteria towards a better understanding of the biodegradation application. Through the utilization of single microorganisms and consortia, researchers were able to biodegrade single pure hydrocarbon components, transform heavy crude oil fractions to lighter fractions, remove heavy metals and reduce viscosity of crude oil.
1. CRUDE OIL: COMPOSITION, PROPERTIES AND DEFINITION
The major elements of crude petroleum oils are carbon (85%-90%) and hydrogen (10%-14%) and the rest are non-hydrocarbon elements, such as sulfur (0.2%-3%), nitrogen (< 0.1%-2%) and oxygen (1%-1.5%), and organo-metallic compounds like lead, vanadium, arsenic, nickel and other metals in traces in parts per million or parts per billion concentration. Inorganic salts of sodium chloride, magnesium chloride and other mineral salts are also accompanied with crude oil from field-wells either owing to formation water or water and chemicals injected during drilling and production operations [1-4]. Crude oil comprises hydrocarbons that are mainly grouped into paraffins (e.g. alkanes), olefins (e.g. alkenes), naphthenes (e.g. cycloalkane) and aromatics (e.g. benzenes) in different proportions. Heavy crude oil contains significant amounts of complex hydrocarbons, such as polynuclear aromatics (PNA) (e.g. polycyclic aromatic hydrocarbons - PAH), alkyl-aromatics, heteroatoms and metal contents, which are more difficult to process. Common hetero-atoms in hydrocarbons are sulfur, oxygen, nitrogen, and metallic atoms [4, 5].
A crude oil with API gravity less than 20° API and usually a sulfur content higher than 2% by weight is considered as heavy oil. Crude oils of API gravity less than 10° API, which is denser than water, are considered as extra heavy oil and these occur in solid or semi-solid state [6]. Conventional oil viscosity may range from 1 centipoise (cP), the viscosity of water, to about 10 cP. Viscosity of heavy and extra heavy oils may range from less than 20 cP to more than 1,000,000 cP [7]. Typically, conventional oils contain 67-97% saturates and aromatics, 2-33% resins and <0.1-12% asphaltene; and heavy oils contain 24-64% saturates and aromatics, 14-39% resins and 11-45% asphaltene [8].
Heavy oils contain substantial amounts of resins and asphaltenes in addition to asphaltogenic acids/compounds, diamondoids and derivative, heavy aromatic hydrocarbons (PNA/PAHs), mercaptans, metal carbenes/organometallic and wax [2, 4, 5]. Asphaltene increases crude oil viscosity and tendency to form emulsions, polymers, and coke [9]. Resins and asphaltenes play a major role in shaping the colloidal nature of heavy oils; hence, the industry’s interest in these heavy oil fractions in particular [10]. Resins and asphaltenes contain numerous heteroatoms as they usually encompass 30-60% of the total sulphur, 70-90% of total nitrogen and 80-90% of the total vanadium and nickel present in a crude oil [11]. Asphaltenes are the heaviest and most polar components of petroleum fluids [12, 13].
2. BIODEGRADATION OF CRUDE OIL AND ENHANCED OIL RECOVERY
Biodegradation is degradation caused by enzymatic process resulting from the action of cells [14]. Bioremediation is defined as the process in which organic wastes are biodegraded under controlled conditions to a safe state or at least to an acceptable level [15]. Bioremediation activities endeavor to increase naturally found degradation rates by adding exogenous microorganisms (bioaugmentation) or stimulating indigenous ones (biostimulation). Bioaugmentation received much attention in recent years as it involves microbes that possess high genetic potential to metabolize a wider spectrum of petroleum hydrocarbons, which is much more effective than biostimulation [16]. Yet, bioaugmentation process may still fail at a field trial stage due to poor bioavailability of pollutants, protozoan predation or competition from native microbiota [16]. Thus, generally bioremediation as a solution that should be evaluated for each individual case of pollution [16]. Crude oil spills that occur during transportation and storage operations, has intensified the oil pollution problems. The persistence of oil may continue even for decades after decontamination [17]. The biodegradability efficiency of the oil components generally decreases in the following order: n-alkanes, branched-chain alkanes, branched alkenes, low molecular weight n-alkyl aromatics, monoaromatics, cyclic alkanes, polycyclic aromatic hydrocarbons (PAHs) and asphaltenes [18]. n-Alkanes which have short hydrocarbon chains are easier to biodegrade than these which have long chains. Rahman et al. [19] showed that nC8-nC11 were degraded completely followed by nC12-nC21, nC22-nC31 and nC32-nC40 with percentage degradations of 100%, 83-98%, 80-85% and 57-73% respectively.
Crude oil contains a large number of non-hydrocarbon components and any alteration in their qualitative or quantitative composition may significantly alter the characteristics of the crude oil. Crude oil biodegradation entails utilization of the crude oil as a substrate for the introduced microbial population [20] and alteration of physical properties through bioproducts [9]. Different reactions that could change the physical properties of heavy crude oil such as oxidizing aromatic ring of a large molecule, chelating metals present in asphaltene aggregates and severing internal bond between asphaltene molecules and large aliphatic chains [13]. The general bacterial mechanisms for accessing petroleum hydrophobic substrates are interfacial accession by direct contact of the cell with the hydrocarbon and biosurfactant-mediated accession by cell contact with emulsified hydrocarbons [21]. Biosurfactants are surface-active chemicals produced by microorganisms in order to adsorb, emulsify, wet, or disperse or solubilize water-immiscible material to use them as a food source [22]. Biosurfactants enhances substrate bio-availability of non-aqueous phase liquids (NAPL), PAHs and other degraded products by increasing oil surface area [23, 24]. Unlike synthetic surfactants, biosurfactants are safe and biodegradable. Many biosurfactants do not comprise true micelles, which may assist direct transfer of the surfactant-associated PAH to bacteria by avoiding nonbioavailability of PAHs inside micelles of synthetic surfactants [25].
The biodegradation concept could also be applied for enhance oil recovery at petroleum reservoir if suitable bacteria, nutrients and reservoir conditions are available. Microbial enhanced oil recovery (MEOR) employs biotechnology to the problems of the petroleum industry [26]. Dwindling light crude oil sources have led explorers to utilize the low quality heavy crude oil resources that are estimated at seven times that of conventional crude oils [27] and investigate different means of heavy oil recovery. MEOR needs little input of energy to produce microbial metabolites in microbial cell factories and its field application does not directly depend on the global crude oil price in comparison to other EOR techniques [28]. MEOR methods are considered more economical and eco-friendly compared to conventional EOR [29]. While the production cost of deep water field with gas and water injection is 3-4 USD/barrel, non-conventional Canadian and Orinoco oil sand is 6 USD/barrel for cold production and 17 USD/barrel for hot/steam production. The production cost of hydrocarbon degrading anaerobic facultative microorganisms is 1.4 - 2.4 USD/barrel [9].
MEOR processes address the same physical parameters as chemical enhanced oil recovery (CEOR). Therefore, they are subject to the same technical difficulties such retention, dissipation and consumption of chemicals in the formation. The essential difference between MEOR and CEOR resides in the method of introducing recovery-enhancing chemicals into reservoir. Accordingly, MEOR should be evaluated on the same basis as CEOR [30]. MEOR technology requires consideration of reservoir properties in terms of salinity, pH, temperature, pressure and nutrient availability. Many petroleum reservoirs have high NaCl concentrations and high temperature, and require the use of microbes that can tolerate these conditions. Since molds, yeasts, algae and protozoa are not suitable due to their size or inability to grow under the conditions present in reservoirs, only bacteria are considered promising candidates for MEOR [31]. According to the type of production problem, microbial delivery processes can be grouped into wellbore cleanup, well stimulation and enhanced water flooding [32]. The biological upgrading goal is to include all activities that make the oil easier to produce and transport, as well as the chemical changes that increase the value of the oil [13]. For biodegradation to succeed as an EOR method, it should reduce concentration of heavy hydrocarbon fractions and increase concentration of the lighter fractions.
3. BACTERIAL BIODEGRADATION ENZYMES
Bacteria with respect to their oxygen requirements are categorized as aerobes, anaerobes, and facultative and each of these categories can be either mesophilic or thermophilic; i.e. capable of life below or above 45°C respectively. The type and yield of metabolic products can be controlled largely by modifications of environmental conditions and nutrients [1]. There are at least 79 bacterial genera that can use hydrocarbons as a sole carbon and energy source, as well as 9 cyanobacterial genera, 103 fungal genera and 14 algal genera that are known to degrade or transform hydrocarbons [33]. Prince et al. [34] listed more than 175 genera of bacteria that are able to grow using hydrocarbons as sole or major carbon source. Bacteria is more versatile than fungi; thus, takes a greater part during biotransformation of hydrocarbons. However, there is no single species which will completely degrade any complex class of hydrocarbons [35].
Reactions involving biopetrochemicals and hydrocarbon degrading organisms were reported by Buehler, Schmid [36]. So far, only oxygen-dependent reactions have been exploited industrially for enzymatic synthesis of fine chemicals from petroleum substrates. In order to utilize bioremediation reactions for biocatalysis or biotransformation, it is necessary to prevent the future degradation and metabolism of the product of choice.
The increase in the rate of substrate transformation may be obtained by controlling enzymatic or regulatory step of the metabolic pathway and by the elevation of the activity of the rate-limiting protein. Enzymes such as oxidoreductase (laccases and cytochrome-P450 mono-oxygenase (CYPs)) are being exploited for degradation of PAHs [37]. Phenotypic traits such as hydrocarbon degradation are frequently carried on plasmids; e.g., Oct, Nah7, dox, TOL, etc [34]. On genes, hydrocarbon catabolic genes: alkM, alkB and xylE genes are responsible for biodegradation of alkanes and aromatic compounds [38] and xylene monooxygenase (xylM), catechol 2,3-dioxygenase (C23O), and benzoyl-CoA reductase (bcr) genes are responsible biodegradation of monoaromatic hydrocarbons [39]. Bacteria could employ multiple alkane hydroxylases including both alkane hydroxylases (pAHs) and cytochrome-P450 enzymes to consume overlapping substrate ranges such as Rhodococcus erythropolis which has up to five pAHs and two CYP153s. However, bacteria could lose its enzyme activity due to operon down-regulation. For example, extended incubation of P. putida GPo1 on n-alkane containing medium caused in the loss of n-alkane oxidizing activity [21].
Xu et al. [40] investigated the distribution of enzyme in different cell parts produced by a microbial consortium and the degradation of naphthalene, phenanthrene, pyrene and crude oil by the osmotic shock method. It was observed that metabolic efficiency of periplasmic, cytoplasmic and extracellular enzymes secreted by a microbial consortium differed according to the utilized substrate. For crude oil, intracellular enzyme activity was higher than extracellular. The authors also investigated the adsorption and uptake of PHCs by live and heat-killed microorganisms. Bioadsorption remained stable for naphthalene, phenanthrene and pyrene whereas crude oil was reduced by dead organisms.
Biodegradation process differs under aerobic and anaerobic conditions. Aerobic biodegradation starts with using molecular oxygen as a co-substrate in mono- or dioxygenase reactions which enable the terminal or sub-terminal hydroxylation of alkane chains or the mono or dihydroxylation of aromatic rings. On the other hand, there are several proposed anaerobic biodegradation mechanisms; however, only addition to fumarate by glycyl-radical enzymes and hydroxylation with water by molybdenum cofactor containing enzymes of an alkyl substituent via dehydrogenase have been characterized in terms of metabolites and enzymes involved [41]. Degradation rates were affected by the temperature range in which the activity occurs. Under normal soil conditions, maximum degradation can be achieved at 30°- 40° C while at fresh water and marine environments, optimum temperature ranged between 20°-30° C and 15°- 20° C respectively [23].
4. EFFECT OF NUTRIENTS AND SALINITY ON BIODEGRADATION
For effective biodegradation process, nitrogen, phosphorus, and in some cases iron are important nutrients [23]. Studies on effects of oil Carbon: Nitrogen: Phosphorus ratios on microbial growth and degradation of oily wastes were not conclusive to devise a fixed recipe since soils, nutrient levels, oil concentrations, and time of incubation parameter varied [42].
Phosphate and nitrate salts are the most common additives which enhance the growth of bacteria effect on the bacterial population [43]. Also higher temperatures, (NH4)2SO4 and K2HPO4 concentrations enhance the growth [44]. However, using excessive nutrient concentrations inhibit the biodegradation activity [23].
To speed up the biodegradation process, yeast extract at 0.01-1% w/v or a similar complex mixture of nutrients may be added to provide a ready supply of compatible solutes such as glycine betaine that can be taken up by the cell, or some other growth factor such as particular amino acid and vitamin. Moreover, co-metabolic degradation of high molecular weight PAHs can be enhanced by the addition of metabolites such as salicylate [45]. Adding surfactants to soils and slurries at high doses (10s to 1000s of mg/kg) at or above the critical micelle concentration (CMC) for promoting PHC solubililization actually inhibits biodegradation due to surfactant toxicity on microbial cells and/or rapid utilization of surfactant in preference to hydrocarbons. Yet, the use of biodegradable surfactants at high concentrations increases biochemical oxygen demand loading and Oxygen, Nitrogen, and Phosphorus nutrient consumption in soils [42]. Addition of chemical fertilizers, wood chips and animal manure could help the biodegradation process. In tests performed by Minai-Tehrani, Herfatmanesh [43], 54% crude oil biodegradation were obtained with the addition of chemical fertilizers and wood chips and 46% crude oil biodegradation with the addition of animal manure.
Since marine oil spills and petroleum reservoir systems are hypersaline in nature, halophilic bacteria may be more suitable for the biodegradation success. Under saline conditions bacteria must synthesize compatible solutes (which causes slow bacterial growth) and overcome decreasing solubility of both oxygen and hydrocarbons as salinity increases [45]. Tam et al. [46] noticed that growth and biodegradation percentages of a consortium made up of two to three different bacterial isolates were not higher than that of individual isolates at low salinity (0 and 10 ppt). However, at high salinity (35 ppt), growth was inhibited and biodegradation of phenanthrene (a tricyclic polycyclic aromatic hydrocarbon) of single bacterial isolates reduced but less inhibitory effect was found on the mixed culture.
5. CASE STUDIES
The mechanisms of biodegradation is complex processes which involve multiple biochemical reactions using one or many bioproducts such as gases, acids, solvents, biosurfactants, biopolymers and biomasses [47]. Biotechnological applications in the petroleum industry includes the biodesulfurization, biodenitrogenation, biodemetallation and biodegradation aspects [29, 31] which represent the biological upgrading processes of heavy oil. The key microbial recovery mechanisms are interfacial tension (IFT) reduction, selective plugging, viscosity reduction, biodegradation and wettability alteration [48]. The factors affecting MEOR process are porosity, permeability, pressure, temperature, dissolved gases, pH, salinity and API gravity [49]. Table 1 highlights some of the bioremediation and microbial enhanced heavy oil recovery through biodegradation cases reported by many researchers.
Microorganism/Metabolite | Results | References |
---|---|---|
Alphaproteobacteria, gamma-proteobacteria and Bacilli. |
Bacterial consortia degraded heavy crude oil from initial C37+ to light hydrocarbons ranging between C11-C27. | [50] |
Biosurfactant produced by B. licheniformis R2 | Enhanced heavy oil recovery by reducing ST/IFT. 37.1% additional oil was recovered. | [51] |
Biosurfactant produced by B. subtilis B30 | Emulsified heavy and light crude oil. The biosurfactant enhanced light oil recovery by 17-26% and heavy oil recovery by 31%. | [52] |
Acinetobacter | 94% biodegradation of saturated hydrocarbon fraction of crude oil after 5 days (industrial scale: 1 ton fermenter) | [53] |
Oil-degrading bacteria and fungi at a wet land | oil in water content decreased from 2-10 mg/l to less than 0.2 mg/l | [54] |
Mycobacterium frederiksbergense & Acinetobacter | Total Petroleum Hydrocarbons (TPH) reduction by consortium, Mycobacterium frederiksbergense and Acinetobacter of 25.1%, 22.3% and 14.5% at sterile conditions respectively and 22.8%, 21.3% and 12.35% at non-sterile conditions respectively. | [16] |
Pseudomonas aeruginosa, Bacillus cereus, Staphylococcus epidermidis, & Micrococcus luteus | P. aeruginosa SBL and B. cereus Z4B-11 degraded 70% and 50% of phenanthrene after one week of incubation, respectively. | [55] |
Burkholderia. | Removal efficiencies of heavy metals from contaminated soils of 44.0% for Zn, 32.5% for Pb, 52.2% for Mn, 37.7% for Cd, 24.1% for Cu and 31.6% for As, respectively. | [56] |
Burkholderia cepacia GS3C, Sphingomonas GY2B and Pandoraea pnomenusa GP3B |
TPH concentration in soil was reduced by 64.4%; however, phytotoxicity and Photobacterium phosphoreum ecotoxicity was increased. | [57] |
Rhodococcus | 65.27±5.63% of crude oil was degraded in 9 days. | [58] |
Garciaella petrolearia | 42% viscosity reduction of heavy oil (2,637 cP at 50°C). | [59] |
Enterobacter cloacea | 76.3% maximum degradation at 0.25% (w/v) heavy crude oil concentration. | [60] |
Pseudomonas & Bacillus | 48% biodegradation of asphaltene by a mixed culture of five strains | [61] |
Serratia, Raoultella & Ochrobactrum | The consortium reduced 37.3% of resins while, aliphatic and aromatic compounds increased by 86.8% and 6.7%, respectively. | [11] |
Indigenous soil microflora | Degraded resins and asphaltenes by 41.5 & 35.0 wt% respectively within 180 days. | [62] |
Bacillus, Pseudomonas aeruginosa, & Micrococcus | 83 to 96% of 2500 mg/L asphaltene was degraded within 21 days at 30° C | [63] |
Geobacillus | Reduced oil viscosity at 50°C by 15.4% to 23.8% at the lab scale and by 1.8% to 14.1% at the field scale. | [64] |
Bacillus licheniformis and Bacillus subtilis | Biotransformed heavier hydrocarbons at heavy crude oil to lighter ones (C12 and C14). B. licheniformis AS5 recovered 16% additional oil at core flooding experiments. | [65] |
Microbial biodegradation process could produce more than one microbial bio-product to facilitate further crude oil degradation. For example, organic acid, bio-gas and polysaccharide (biopolymer) bio-products produced by the bacterial strain Streptococcus sp. BT-003 aided biodegradation and usage of crude oil as the only source of carbon. The bio-products caused crude oil emulsification and crude oil viscosity reduction from 8000-15000 mPa·s to 50-250 mPa·s [47]. Produced glycolipid biosurfactant, by Bacillus methylotrophicus USTBa bacterial strain, lowered surface tension of water to 28 mN/m at CMC of about 38 mg/L and showed emulsification activities and enhanced crude oil biodegradation [66].
Employing more than one bacterial strain to enhance biodegradation is an attractive option. Rahman et al. [67] achieved higher bacterial growth and crude oil degradation by mixed bacterial consortium (78% degradation of BH crude oil at 1% crude oil concentration) than by individual bacterial cultures (ranging between 66% degradation by Pseudomonas sp. DS10-129 and 41% degradation by Flavobacterium sp. DS5-73 at the same concentration. Cameotra, Singh [68] reached more than 98% crude oil degradation when nutrient mixture and a crude biosurfactant preparation were mixed with a consortium.
Tavassoli et al. [61] demonstrated 48% biodegradation of asphaltene by a mixed culture of five strains exceeding the highest ever reported asphaltene biodegradation result (35%). Generally, microbial growth on hydrocarbons at anaerobic conditions is slow in comparison to microbial growth at aerobic conditions [69]. However, that does not eliminate the possibility of having comparable results under aerobic and anaerobic conditions evidenced by Streptococcus sp. BT-003 strain [47].
Bacterial strain Garciaella petrolearia TERIG02 showed preference to, unlike the majority of microbes, toxic asphalt and aromatics compounds and was able to reduce viscosity of heavy oil (2,637 cP at 50°C) by 37% when asphalt was the only source of carbon and by 42% when molasses was added [59]. Bio-gases and bio-solvents were responsible for the decreased surface and interfacial tensions and emulsification processes. The authors added that bio-mass by itself may aid in increasing sweep efficiency at reservoir applications. Enterobacter cloacea ERCPPI-1 bacterial strain through utilizing heavy crude oil as the sole carbon source reached a maximum of 76.3% of degradation at 0.25% (w/v) concentration of heavy crude oil after 21 days of incubation and it produced biosurfactant, with high oil spreading and emulsification properties. Nevertheless, at 10% heavy crude oil concentration, degradation percentage by the strain decreased to 19.1% only [60].
Huang et al. [64] isolated KSH-1 (unidentified), KSH-2 (Geobacillus sp.) and KSH-3 (Geobacillus sp.) from produced water of Unit Jian-12, Huabei heavy oil field, China (204 mP.s at 50°C) where water flooding no longer recovered residual oil efficiently. The three strains were tested at laboratory and field scales (Table 1). At a lab scale, each bacteria biodegraded Huabei heavy crude oil and produced biosurfactant. At a field level, a two-cycle MEOR field trial was conducted as per the specified composition of MEOR injection. However, unexpected biomass and biofilm were produced under in-situ conditions, which caused injection profile modification blocking a thief zone. Injection was continued for about 25 days followed by 10-day shut-in period of injection wells. The total oil recovery increased by 1.5% and the average additional cost was $11.31 per barrel.
Bachmann et al. [9] reported hydrocarbon-degrading and extracellular biosurfactant producing Bacillus subtilis strains degraded normal alkanes higher than C27 and increased percentage of less than C25 normal alkanes under anaerobic conditions. Besides, biotransformation could aid in breaking down precipitated paraffinic and asphaltic fractions at reservoir conditions thus restoring permeability. Bacterial strains could utilize n-alkanes which are solid at ambient temperature (e.g. paraffin waxes and C18 and longer) such as Acinetobacter sp. M-1 which metabolized C13-C44 as a sole carbon source [21]. To get crudes and fuels into perspective in terms of carbon number, crude oil ranges from C2 to C60+, gasoline fuel from C5 to C11, kerosnene fuel from C6 to C16, diesel fuel no.2 from C8 to C21, jet fuels from C5 to C18, heating oil no. 2 from C8 to C24 and motor/lubricating oil from C10 to C24 [42].
Unlike n-alkanes, highly branched alkanes, cycloalkanes, 4 to 6 ring condensed aromatics, alkylated thiophenes and dibenzothiophenes are harder to biodegrade and are partially metabolized or are completely recalcitrant [42]. The reactivity PAHs decreases in the following order: n-alkanes, Isoalkanes, cycloalkanes then aromatics [62]. Rhodococcus erythropolis bacteria that could biotransform PAHs, polychlorinated biphenyls and dibenzothiophenes [70].
Karim et al. [71] tested biodegradation on low wax, low sulfur, low asphaltene and low pour point crude oil of 17-20 API° at lab and field levels. Biodegradation study indicated complete removal of normal/branched alkanes and partial removal of aromatics due to in-situ alteration without damaging Bokor’s sandstone formation. However, the crude oil was already biodegraded before the microbial treatment and the API gravity did not change significantly even after two months after the five weeks shut-in of the three tested single well tests [71]. This minor improvement in oil quality did not affect the bulk properties and it was observed at the gas chromatographic level by about 2% increase in abundance only.
Bacterial isolates, from our ongoing study, showed almost complete degradation of heavy crude oil (13.3° API) within 36h incubation at 40° C at aerobic conditions (Fig. 1). The figure shows the clearance zones observed at well assays test carried for 3 bacterial isolates: AS1, AS3 and AS11 (GenBank NCBI accession numbers: KJ729814, KJ729816 and KJ729824 respectively) [65]. 16% additional oil was recovered at core flooding experiments.
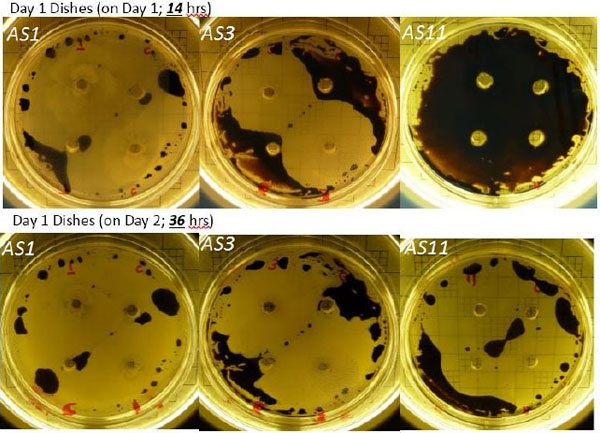
A thorough survey on MEOR field trials from across the world was conducted by Maudgalya et al. [72] where 407 MEOR tests and field trials were reviewed. On biodegradation, 29 out of 34 (85%) trials were successful and the authors expressed if consistent success can be achieved as some tests have shown, oil biodegradation can be especially useful for heavy oil recovery.
Table 2 shows some of the general screening criteria used to assess the possibility of the MEOR application. These criteria by the different authors are similar on some of the parameter; nevertheless, there are some parameters with noticeable range differences. The application of biodegradation process is not limited to a certain predefined range but rather controlled by the ability of involved microorganisms.
Lazar* | DOE* | Reviewed Projects Range* | 24 Norwegian Fields Range* | Jackson et al. [73] | Karim et al. [71] | |
---|---|---|---|---|---|---|
Porosity (%) | ≥ 20 | - | 8 – 32 | 11 – 35 | - | ≥ 10 |
Permeability (mD) | ≥ 150 | > 50 | 0.1 – 5,770 | 1 – 20,000 | 50 to 100 | ≥ 20 |
Reservoir Temperature (°C) | ≤ 70 | < 80 | 19 – 82 | 61 – 155 | < 60 – 70 | < 132 |
Salinity (g/l) | ≤ 150 | ≤ 150 | 1.4 – 104 | 14 – 273 | ** | < 100 |
Oil Viscosity (cP) | 5 – 50 | - | 3 – 50 | 0.1 – 4.83 | < 500 | 5 – 50 |
Reservoir Depth (m) | - | < 2,347 | 122 – 2,103 | 1,300 – 4,208 | - | - |
pH | - | - | - | - | 5-9 (6-8 ideal) | - |
Down-hole Pressure | - | - | - | - | < 3000 psi | - |
API Gravity (°API) | - | - | - | - | - | 10 – 50 |
Paraffin Wax (%) | - | - | - | - | - | ≥ 3 |
Previous Biodegradation | - | - | - | - | - | Little or none |
Pressure Gradient (psi/ft) | - | - | - | - | - | > 0.1 |
Water Cut (%) | - | - | - | - | - | 10 – 50 |
CONCLUDING REMARKS
Microbial biodegradation of heavy crude oil holds a promising opportunity to remove or at least safely alleviate the environmental impact of oil spill cases. Biodegradation as a secondary objective could also satisfy energy needs through utilizing heavy deposits and avoiding the high costs associated with EOR technology. The main obstacle so far in the research of heavy oil biodegradation is finding efficient microbes that could degrade the heavy petroleum components (asphaltenes and resins) and withstand harsh conditions in a relatively short incubation time. Future research on the subject of heavy crude oil bioremediation and biodegradation application at petroleum fields are needed to fully utilize the opportunity in hand.
CONFLICT OF INTEREST
The authors confirm that this article content has no conflict of interest.
ACKNOWLEDGEMENTS
Declared none.